11 Module 11: Brain and Behavior
In section 10.1, we noted that genes are responsible for building all of the cells in our body. In this module, we will introduce you to many of the cells and groups of cells that genes build in the nervous system.
The individual cells in the nervous system are called neurons, cells that generate and transmit electrochemical signals. Neurons are the basic cells of the nervous system, including our brains. Our genetic blueprints also set up the organization of those neurons into the different divisions of the nervous system and the specific parts or functional areas within the brain.
Module 11 is divided into three sections. Section 11.1 describes the electrochemical activity that takes place in an individual neuron and allows neurons to communicate with each other. It also explains a bit more about the “astonishing hypothesis” and lays out the divisions between the parts of the nervous system. Section 11.2 is devoted to the brain; it describes the functions and locations of several of the parts that are most important in human behavior and mental processes. Section 11.3 brings together the information from the previous two sections by returning to the communication process between neurons. The section describes neurotransmitters, the chemicals that carry signals between neurons throughout the nervous system. It concludes with a short introduction to neuropsychopharmacology, the understanding of brain and behavior through the discovery of the neural actions of drugs.
11.1 Neurons and the Nervous System
11.2 The Brain and Behavior
11.3 Neurotransmitters and Neuropsychopharmacology
READING WITH A PURPOSE
Remember and Understand
By reading and studying Module 11, you should be able to remember and describe:
- Action potential and resting potential: dendrites, cell body, axon, myelin (11.1)
- Neural communication: terminal button, vesicle, neurotransmitter, synapse, receptor site (11.1)
- Central and peripheral, somatic and autonomic, sympathetic and parasympathetic nervous systems (11.1)
- Major parts and functions of hindbrain: medulla, pons, reticular formation, cerebellum, thalamus (11.2)
- Major parts and functions of forebrain: hypothalamus, pituitary gland, amygdala, hippocampus, limbic system, cerebral cortex, frontal lobe, parietal lobe, occipital lobe, temporal lobe, primary motor cortex, prefrontal cortex, primary sensory cortex, primary visual cortex, primary auditory cortex (11.2)
- Major functions and location of midbrain and corpus callosum (11.2)
- Methods of discovering functions of the brain: case studies, animal research, electroencephalogram, positron emission tomography, functional magnetic resonance imaging (11.2)
- Common neurotransmitters: endorphins, cannabinoids, serotonin, GABA, acetylcholine, norepinephrine, dopamine (11.3)
- Neuropsychopharmacology: Agonists and antagonists, reuptake (11.3)
Apply
By reading and thinking about how the concepts in Module 11 apply to real life, you should be able to:
- Come up with your own examples of situations in which your sympathetic and parasympathetic nervous systems were active (11.1)
- Predict the behavior changes that might result from a brain injury or disorder (11.2)
Analyze, Evaluate, or Create
By reading and thinking about Module 11, participating in classroom activities, and completing out-of-class assignments, you should be able to:
- Evaluate the Astonishing Hypothesis in light of the computational theory of the mind (11.1)
- Propose potential agonistic or antagonistic mechanisms of specific substances and drugs (11.3)
11.1 Neurons and the Nervous System
Activate
- What is your opinion of the “astonishing hypothesis”? Are you comfortable with the idea that everything you do and are can be traced to the electrical and chemical activity in your nervous system?
- Have you ever thought about how computer programs really work? Specifically, how does the computer translate the instructions from a computer programming language into a set of electrical signals that carry out the instructions?
The cognitive revolution (Module 9) showed us how mental processes, such as intentions, desires, and consciousness, could be seen as the manipulation of information within the brain. Thus it helps us begin to understand the “astonishing hypothesis,” the idea that everything we are and do starts with electrochemical processes within and among nerve cells. Researchers have called it the computational theory of mind. The brain receives input from the world through the sensory organs (details in Module 12). The input is translated into neural signals that correspond to the information from the world; then, the neural signals are transmitted to other parts of the brain for more processing.
Although you do not need to know all of the details of this process, a few observations and facts can help you to see how these neural signals might work to create a complex thinking system, such as a human brain:
- As you will see in this section, a neuron is very much like a little switch; it is either on or off.
- Computer programs and mental processes are both computational procedures, in which information is manipulated.
- Any computational procedure can be expressed as a series of little on/off signals.
This last point was proven mathematically by the mathematician Alan Turing in 1936. Think of the most complicated computer program you can imagine (for example, a complex video game or a program that can recognize speech, a very difficult task). Although the program was written in a programming language, the commands are actually translated into a series of on/off signals, and that is what the computer executes. Of course, for a complicated computational procedure, it would be a very long and very complex sequence of signals. Because our mental processes are probably among the most complicated computational procedures in the universe, the sequence of on/off signals, provided by our neurons, would be extremely complex. These procedures are so complex that researchers still are not very close to figuring out what the sequence is, by the way.
Neural Signals
Module 5 briefly introduced you to the parts of a neuron and described the process of ion exchange that constitutes neural activity. In this section, you will get many more details about this process. Although the process is complicated, remember that what the neuron is accomplishing is actually quite simple; it is either turning on or staying off, just like the signals or switches that constitute complex computer programs, or the light switch in your room.
Recall that the three main parts of a neuron, for our purposes, are the dendrites, cell body, and axon. The typical neuron has many dendrites, one cell body, and a single axon (there are other types of neurons but we can learn the main ideas by focusing on this typical type). The purpose of dendrites is to receive incoming signals; the purpose of the axon is to carry a neural signal away from the neuron. (See Figure 11.1)
There are three main processes involved in neural activity:
- The initiation of an electrical signal in a neuron
- The movement of that electrical signal through the neuron’s axon
- The transmission of the signal, in chemical form, to other neurons
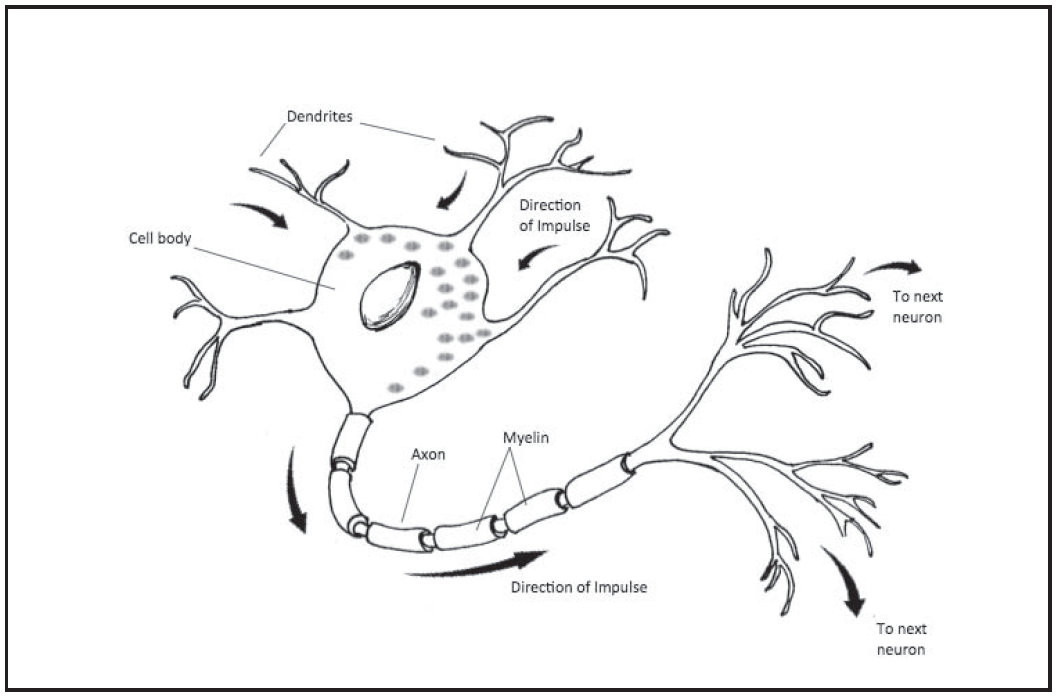
axon: the single “tube” in a typical neuron that carries an electrical signal to other neurons
dendrites: the many branches that each neuron has; dendrites receive incoming information from the outside world and from other neurons
Resting Potential and Action Potential: Initiation of the Signal and Its Movement Through the Axon
A neuron receives excitatory and inhibitory signals at its dendrites and cell body; the signals come from many other neurons or from stimulation from the outside world. An excitatory signal is one that is instructing the neuron to pass along the signal. An inhibitory signal is one that is instructing the neuron not to transmit a signal. (Think of the excitatory signal as telling the neuron to “turn on,” and the inhibitory signal telling it to “stay off” if you’re thinking of the switch analogy). The neuron collects all of these signals and adds them together. If there are enough excitatory signals, the neuron fires and generates an action potential.
The most important concepts for you to understand in the neural signaling process are the action potential and resting potential. Potential is another word for voltage, or potential difference. It is basically a difference in electrical charge between two areas. The electrical charge is the result of the location of electrically charged particles called ions. There is a potential, or potential difference, because there is a different concentration of positive and negative ions inside and outside of the axon of a neuron.
The resting potential is the voltage on the inside versus the outside of the neuron when it is at rest (when it is “off”). The action potential is simply a voltage, or an electrical charge, that travels down the length of a neuron’s axon (when it is “on”). It begins at the part of the axon nearest to the cell body and makes its way bit by bit to the end of the axon.
Why are ions crucial to action potentials and resting potentials? Think about the concept that opposites attract and likes repel. A positively charged ion is attracted to a negatively charged ion and repelled from another positively charged ion. Similarly, a negatively charged ion is attracted to a positive one and repelled from another negative one.
Let’s delve a little deeper into the application of this concept to neural activity, so you can fully understand action and resting potentials. Neurons are floating in fluid, and they have fluid inside of them. There are electrically charged ions in both fluids. In the fluid on the outside of the neuron, there are positive ions, specifically sodium (Na+), and negative ions, specifically chloride (Cl-). There are more sodium ions than chloride ions, so the fluid outside of the cell has an overall positive electrical charge. When the neuron is at rest (i.e., resting potential), inside the cell there are positive ions, (K+), and negative ions, chloride (Cl-) and other anions (negatively charged particles, essentially proteins). Because of the chloride and the other anions, the fluid inside the neuron has an overall negative electrical charge.
- So, to summarize so far: Lots of sodium (Na+) is outside, lots of chloride and protein are inside (both negative) when the neuron is at resting potential.
Remember: Opposites attract and likes repel. Negative ions will try to move away from other negative ions and toward positive ions. Positive ions try to move away from other positive ones and toward negative ones. The effect is that the negative ions are trying to get out of the neuron and the positive ions are trying to get in. At the same time, particles have a tendency to move from areas where they are highly concentrated to areas of low concentration, a process called diffusion. So, sodium, being highly concentrated on the outside is trying to move inside because of diffusion as well as the attraction to the negative particles. The surface, or membrane, of the neuron is what limits this activity that the particles tend toward, resulting in the electrical potential, specifically, the resting potential.
- During the resting potential, the neuron has an overall negative charge, as there are more negative ions inside, and more positive ions outside. At the same time, the negative are trying to get out while the positive are trying to get in.
When the action potential begins, the surface of the axon nearest to the cell body changes. Basically, some channels open up to allow ions to move more freely into the axon. Because the sodium ions are attracted to the inside of the neuron, they immediately rush into the now opened section. This is the essence of the action potential; it is a positive electrical charge that is caused by the influx of sodium ions into one tiny section of the axon. What happens next is the same type of channels open in the section of the axon a little farther out, allowing a new rush of sodium into this new section. An instant later, new channels that will allow ions to flow out open up in the first section of the axon. Positive ions, this time potassium, can now be forced out of the axon by the abundant sodium ions surrounding them. Thus, the first section of axon has its electrical charge returned to normal. The process then repeats itself again and again down the entire length of the axon. (See the four-part Figure 11.2, beginning below.)
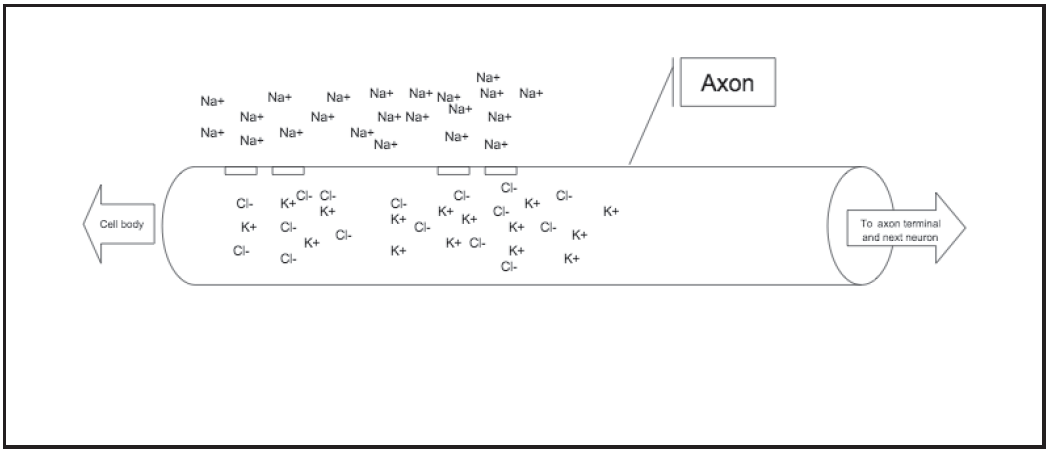
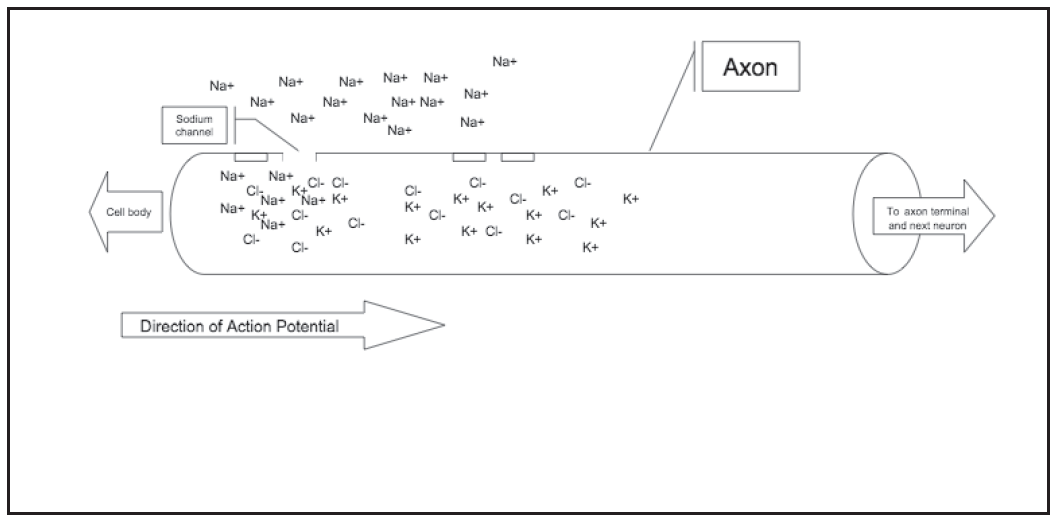
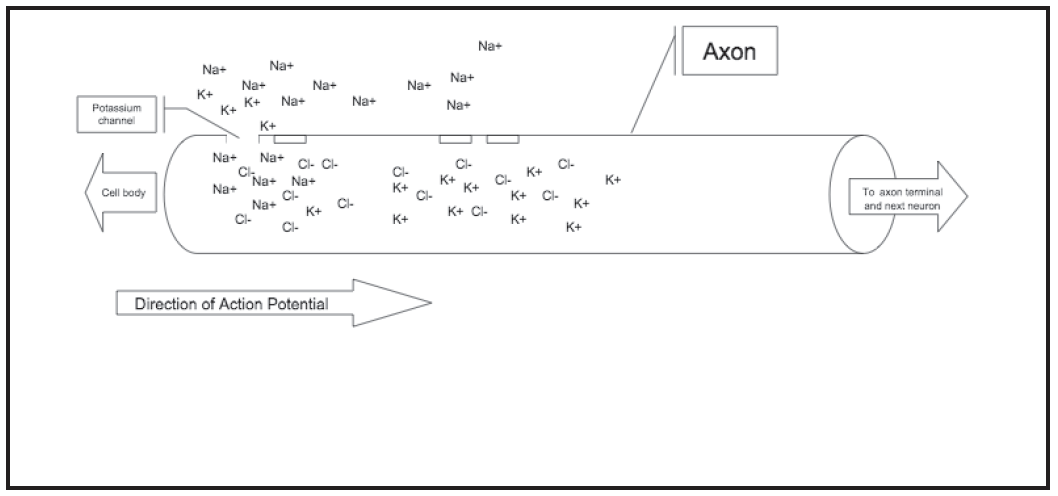
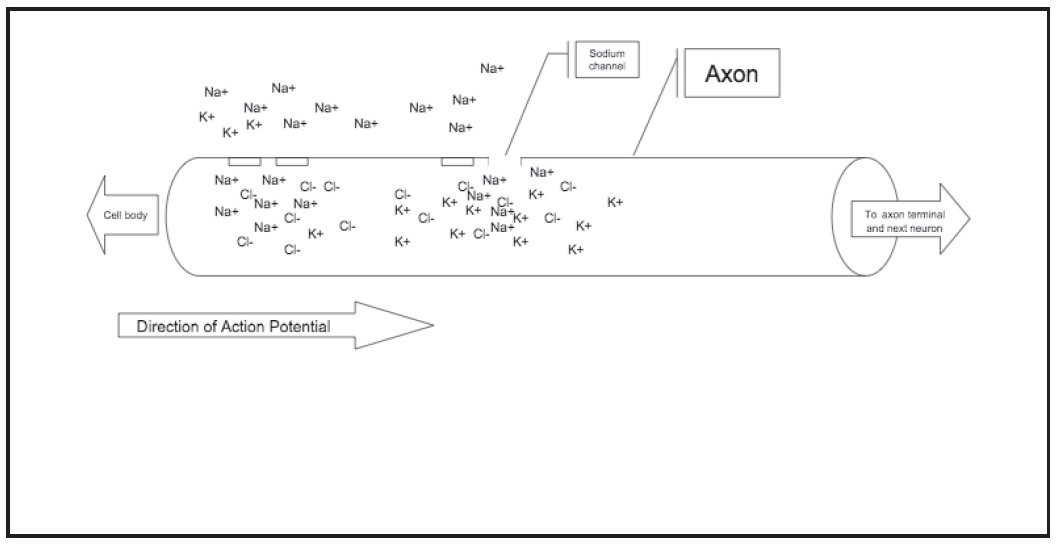
Let us pause briefly to put the process so far together:
- Dendrites and the cell body of a neuron receive excitatory and inhibitory signals, mostly from other neurons.
- If enough excitatory signals are transmitted to the neuron, it begins an action potential at the part of the axon nearest to the cell body.
- The action potential proceeds as sodium ions move into and potassium ions move out of the axon; the action potential travels one microscopic section at a time down the length of the axon.
anion: a negatively charged particle
diffusion: the tendency for particles to move from areas of high concentration to areas of lower concentration
ion: an electrically charged particle
action potential: an electrical signal (voltage) that travels down a neuron’s axon; it results from the movement of positive ions into and out of the axon
resting potential: the voltage of a neuron when it is at rest; it results from positive ions outside and negative ions inside the neuron
excitatory signal: a signal entering at a neuron’s dendrites or cell body instructing the neuron to transmit its own signal
inhibitory signal: a signal entering at a neuron’s dendrites or cell body instructing the neuron to not transmit its own signal
An action potential is quite slow. Ions must flow in sequence into and out of an axon throughout its entire length (an axon of a neuron involved in movement can be several feet long). Fortunately, many neurons have a method that speeds up the process. Specifically, other cells in the nervous system, called glia, form a substance called myelin, which wraps around the axon and joins together some of the microscopic sections to form larger sections (see Figure 11.1 above). The positive ions that make up the action potential quickly float through each myelinated portion of the axon, and the action potential itself takes place only at the breaks between the myelin sections (the sections where the action potential occurs are called nodes of Ranvier).
By the way, glia are among the most interesting topics to current neuroscientists. For many years, researchers thought that glia served little purpose other than to protect, provide nutrients for, and hold together the neurons of the brain (glia means “glue”). Recently, researchers have discovered that glia actually participate in the signaling that we thought was unique to neurons. Currently, many researchers are trying to discover the full contribution of glia to the functions of the nervous system (see Module 14).
glia: types of cells, other than neurons, in the nervous system
myelin: a substance that covers many of the brain’s neuron’s axons; it protects the axon and speeds up the action potential by allowing it to jump from one non-myelinated section to the next.
Neural Communication: Transmission of the Signal to Other Neurons
When the action potential reaches the end of the axon, it must be transmitted to other neurons in order for its signal to be carried throughout the nervous system. This communication between neurons takes place at what is called a synapse, the area where two neurons come together. A synapse is where the end of the axon of one neuron is situated very close to (but not touching) a dendrite or the cell body of a neighboring neuron. Neural communication takes place when chemicals called neurotransmitters are released from the axon, float across the small space between the two neurons, and land on the dendrite or cell body of the neighboring neuron.
A few details are necessary to understand the process well. Axons end with their own little branching sections, and each tiny branch ends with a slightly swelled area called a terminal button. Neurotransmitters are stored in tiny spaces called vesicles in the terminal buttons. When an action potential reaches a terminal button, it causes the vesicles to open and release their neurotransmitters into the synaptic gap between the two neurons. The neurotransmitters float across the gap and land on receptor sites on the dendrites or cell body of the neighboring neuron. Then, the receptor sites open up to allow ions to float into or out of the neuron. These ions will cause tiny electrical charges, or potentials, in the second neuron. In this way, the signal is sent from the first to the second neuron.
Some neurotransmitters are excitatory; other neurotransmitters are inhibitory. A receiving neuron must collect all of these excitatory and inhibitory signals and decide whether or not it will generate its own signal. We hope that this sounds familiar. This, of course, is the input collection process that we described at the beginning of this section. There are many different neurotransmitters, and each one can only influence certain receptor sites, much like a key that can only fit certain locks. Keep in mind that an axon branches off into many terminals (sometimes thousands), each of which forms a synapse with a neighboring neuron, so a single neuron releases very many neurotransmitters to many different other neurons.

synapse: the tiny area between two neurons, where neural communication takes place
neurotransmitter: the chemicals that carry a neural signal from the axon of one neuron to the cell body or dendrites of a neighboring neuron.
terminal buttons: the end section of axon branches, from where neurotransmitters are released.
vesicles: the storage sites for neurotransmitters in the axon, before they are released.
receptor sites: the sections on cell bodies and dendrites where neurotransmitters land, thus completing the transmission of a signal from one neuron to another.
Stimuli for Neural Activity
One of the more confusing aspect of the process of neural communication may be the question of how or where the whole thing starts. We mentioned that our target neuron is collecting signals mostly from other neurons, which are collecting signals from other neurons, which are collecting signals from other neurons, and so on. So where does the process start?
Often the first neuron in the process is a sensory neuron, one that receives input from the outside world. For example, your eye receives light, which is transformed into neural signals, which are recognized as a table or some other thing as the signal flows through the brain.
What about when input from the external world does not seem to begin the process? Where do spontaneous, random-seeming thoughts come from?
Imagine taking a shower in a health club locker room. As you turn off the water and begin drying your face, the distinctive odor of the industrially washed towel instantly reminds you of summer camp when you were in middle school. Then middle school reminds you of high school, which reminds you of your favorite teacher, who hired you to work as a lifeguard at a community pool during one summer. After reminiscing about your high school summers, and in particular, the attractive person with whom you used to flirt in the guard station, you looked up and wondered why you were thinking about them. This kind of thinking, known commonly as a stream of consciousness, is not random, although it might seem like it is. Each successive thought was clearly connected to the thought that preceded it. Imagine, then, a set of neural signals that leads from one thought to the next. The only thought that is not preceded by another one is the first. What preceded that thought? The odor of the towel. So, the whole stream of consciousness began when the external environment was perceived. That perception led to a thought (the initial reminding), and the whole stream of thoughts took off from there. Pay attention to it yourself sometime. Work backwards from your current stray thought. What made you think of it, what made you think of the previous thought, and so on? You may be surprised to discover how often the stream of thoughts can be traced back to some stimulus in the environment. Many thoughts that seem random are not.
What about when something really does just pop into your head, however? How does that happen? Many of these events can probably explained by the way memories are stored and retrieved. When an event occurs and is stored in memory, many properties of the event are stored with it (colors, odors, sounds, emotions, physical feelings, and so on). Any of these properties may serve as the entry point into the memory, that is, as a retrieval cue (Module 5). For example, imagine that you receive the best grade on your next psychology exam that you have ever gotten, and the news puts you in a very happy mood. Several weeks later, a new event puts you in the same mood; the repetition of the mood reminds you of the exam. If you are reminded of some event without realizing what did the reminding, the consequent thought will seem as if it just popped into your head.
A great deal of mental processing goes on outside of conscious awareness. Often the results of that unconscious processing seem as if they just popped into your head. It is also possible, though, that sometimes a thought really is random. Neurons fire spontaneously and randomly throughout the day. Most of the time, this random firing has no effect on our thinking. Recall that in order for a neural signal to have an effect on another neuron, the signal must be combined with the signals from many other neurons. Occasionally, however, the results of random neural firing may lead to sufficient stimulation to generate a series of signals that can lead to a conscious thought.
The Organization of Neurons into the Nervous System
Just as few thoughts are truly random, neurons are not joined together randomly. There are specific pathways and clusters of cells throughout the nervous system. They make up key parts of the brain and important pathways for information flow throughout the nervous system (for example, see Module 12 about vision pathways).
In addition, there are some major divisions in the nervous system. The most basic division is between the central nervous system and the peripheral nervous system. The central nervous system is simply the brain and spinal cord, basically command central for the rest of the nervous system. The peripheral nervous system is the part that runs through the rest of the body; it is divided into the somatic nervous system and the autonomic nervous system. The somatic nervous system controls many of the muscles of the body; it consists primarily of neurons for sensation, sensory neurons, and for body movement, motor neurons. For example, as you sit in the library studying for an upcoming exam, sensory neurons within the somatic nervous system relay information about pressure and tension from the lower parts of your body and your back muscles to the central nervous system; you are uncomfortable from sitting in the same position for three solid hours. Then, the central nervous system might “answer,” sending information via motor neurons that lead you to shift in your seat (or get up) so that you are more comfortable.
The autonomic nervous system controls the glands and internal organs; it is subdivided into the sympathetic and parasympathetic nervous systems. The sympathetic nervous system causes several responses throughout the body that increase arousal and prepare it for activity. The arousal that comes from the sympathetic nervous system is commonly known as the fight-or-flight response, a set of responses that prepare the body to meet or escape from a threatening situation. The parasympathetic nervous system reverses those responses, calming the body back down. Imagine that you are running late for work one morning and driving a bit over the speed limit. Suddenly, up ahead, you see a police car on the side of the road. Instantly, your sympathetic nervous system kicks into gear and your fight-or-flight response begins. Your digestive system began to shut down: your mouth gets dry and you get the “butterflies in the stomach” feeling. Blood that had been flowing to your digestive system is diverted to the large muscles of your arms and legs, the better to fight or flee from the imminent danger (free advice: not a good idea with a police officer). Your palms get sweaty to improve your grip (again, part of the fight response). As you get closer to the police car, you discover that it is empty. It is a decoy car, planted there to frighten violators into reducing their speed. Just as instantly, the fight or flight response begins to reverse as the parasympathetic nervous system takes over to return your body to its normal, unstressed state. As you will see in Modules 20 and 28, this fight or flight response plays important roles in stress and health, and in emotions.
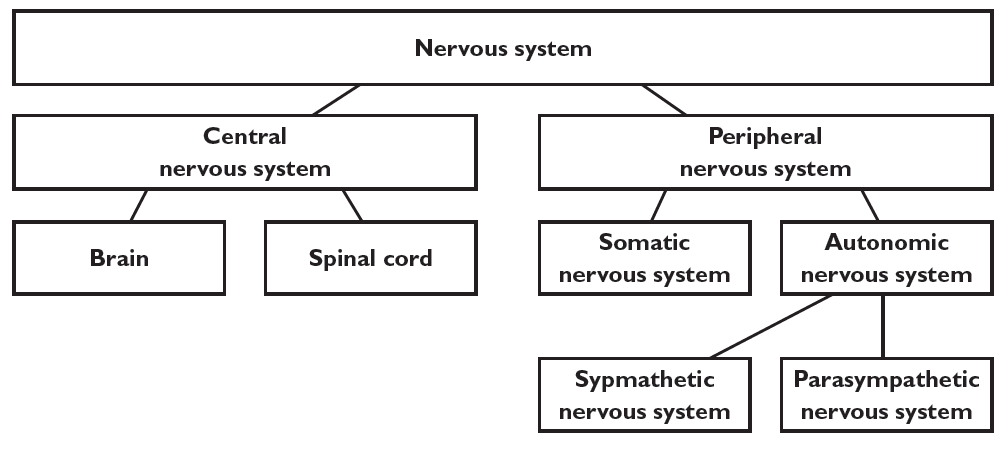
central nervous system: the brain and spinal cord; the command center of the nervous system.
peripheral nervous system: the parts of the nervous system that run throughout the body (everything except the brain and spinal cord).
somatic nervous system: the part of the peripheral nervous system that controls the skeletal muscles
sensory neurons: neurons that receive input from the outside world and send sensory information to the brain
motor neurons: neurons that are responsible for producing movement
autonomic nervous system: the part of the peripheral nervous system that controls the glands and internal organs
sympathetic nervous system: the division of the autonomic nervous system that arouses the body
flight-or-flight response: the common name for the set of arousing responses produced by the sympathetic nervous system; they are designed to prepare the body to face some physical danger by fighting it or fleeing from it.
parasympathetic nervous system: the division of the autonomic nervous system that calms the body down
Debrief
- What is the easiest part of the whole action potential/neural communication process for you to understand? What is the hardest part? How would you explain the parts that you understand well to someone who is having difficulty understanding?
- Come up with your own example of an event during which your sympathetic and parasympathetic nervous systems were at work. Can you recognize the fight-or-flight reactions?
11.2 The Brain and Behavior
- Draw a sketch of the human brain. From memory, add any labels you can showing the names of different areas of the brain or their functions.
- Have you or anyone you know had a brain disorder or injury? Where was it located? What was its effect on mental processes, emotion, or behavior? Was there a way to compensate for the disorder?
One of the most important and interesting discoveries about the brain has been its plasticity. In short, the brain can reorganize itself as a consequence of experience or damage. For example, taxi drivers have a larger part of the brain that appears to be related to navigation skill than non-taxi driving adults, and the amount of brain area is related to experience as a driver (Maguire et al., 2000).
Sometimes, the plasticity can be dramatic. In a feat of nearly Frankensteinean proportions, researchers were able to rewire the brains of ferrets so that visual information was sent to the brain area that was supposed to process sounds, and vice versa (Sur et al., 1999). The ferrets’ brains were able to reorganize so that the animals were able to function correctly.
Although no one has attempted such a dramatic demonstration with a human brain, there are striking examples of human plasticity as well. Perhaps the most amazing example is when an operation called a hemispherectomy is performed. This operation, the actual removal of one hemisphere (half) of a brain, has been used on very rare occasions as a treatment for severe and degenerative seizures. Research has shown that the operation can substantially reduce symptoms, and patients can function quite well, in many cases as well as people who have intact brains (Moosa et al., 2013; Vining et al., 1997). Recent research compared brain scans of six hemispherectomy patients to a large group of healthy controls. They found that several functional areas of the brain had stronger interconnections for the 6 patients than for the normal controls, as if their brains compensated for the missing halves by increasing connections in the remaining hemisphere (Kliemann et al., 2019).
When you first look at a brain, you see a lumpy, light grayish-brown, wrinkled mass, with few discernible parts. At first, then, it seems plausible that the brain might be infinitely plastic. A great many people do believe that the brain can basically reorganize itself without limit. With a little careful examination, however, you can begin to notice that there are separate sections. For example, on the surface of the brain, some of the wrinkles look larger than others, and some of the lumps are more pronounced than others. These separate areas are recognizable on any brain, and they are completely unrelated to any damage or experience. So, without denying that the brain can change itself, you must realize that the brain has a very intricate structure, very specific parts biologically determined to fulfill specific functions.
Just as a skilled radiologist can recognize what appear to the untrained eye to be unintelligible specks on an x-ray of the body, you can learn to recognize these different areas in the brain. If you intend to be a neuroscientist, you’ll definitely need to acquire this skill. It is also handy for psychologists, given today’s emphasis on biopsychology. But even if you never have a professional need to recognize the brain’s features, we think that you will find them interesting. And although we hope you never have this experience, somebody you know might someday have a brain disorder or injury that will make your study of the brain’s geography entirely relevant.
In this section, we will introduce you to a few of the main parts of the brain and give you some information about their functions. This is a useful backbone of knowledge for your study of the rest of the book. When appropriate in other sections, we include other descriptions of brain parts and functions as they apply to various psychological phenomena.
Brain Areas
The most basic distinction in the brain is between the hindbrain, midbrain, and forebrain. As we are sure you can guess from the names, these parts describe the locations of the areas in the brain. These locations are difficult to see in the human brain, however. The forebrain in humans is so large it covers the midbrain and part of the hindbrain.
Each of the three major areas of the human brain incorporates several smaller brain structures. Brain structures that are located close to each other often have similar or complementary functions. The individual sections that make up the hindbrain are largely devoted to basic survival functions. Many midbrain sections are important for processing sensory information and movements. Finally, the forebrain contains structures that further process sensory information, regulate our emotions, and carry out our higher intellectual functions.
hindbrain: the structures of the brain most closely related to basic survival functions
midbrain: structures of the brain closely related to processing sensory information and movements
forebrain: structures of the brain that process sensory information, regulate emotions, and carry out higher intellectual functions
Hindbrain and Midbrain
The hindbrain is composed of the following four individual brain parts: medulla, pons, reticular formation, and cerebellum. (See Figure 11.5)
- As the spinal cord reaches up into the skull, it begins to widen. The medulla is the wider area at the very base of the brain; it is essentially the first brain part that is distinct from the spinal cord. The medulla controls basic survival-type functions, such as heart rate and breathing.
- Just above the medulla is a second, more distinctly bulging area, the pons, which functions a bridge, transferring information between the brain and the spinal cord.
- Stretched inside of the medulla and pons is an area called the reticular formation, a brain area important for attention and arousal.
- The cerebellum is the part that looks like a miniature brain (cerebellum means “little brain”); it is tucked behind the medulla and pons. Do not be fooled by the name, “little brain,” however. The cerebellum contains more than 50% of the brain’s neurons despite being only 10% of the brain’s total volume. So obviously, it is an extremely important part of the brain. The cerebellum helps us with posture and balance, and it helps us to learn and coordinate voluntary movements (Byrne, 1997; Leiner, Leiner & Dow, 1995). The cerebellum is quite large in humans compared to other animals, suggesting that it has an important role in cognition. The cerebellum appears to be important for learning higher-order cognitive skills, such as reasoning, by making complex thinking procedures more routine (Preuss, 2000).
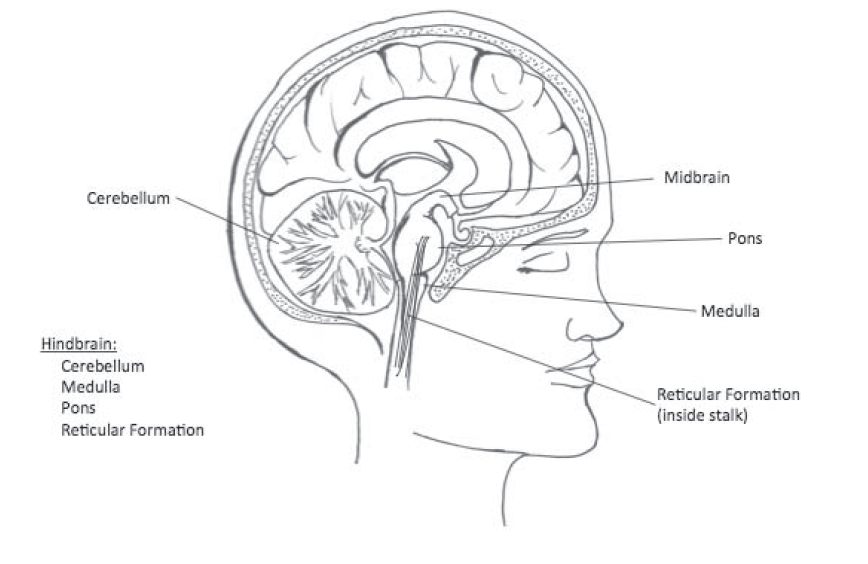
Although the midbrain does contain several individual parts, we will keep things simple for now by not subdividing it. In general, the midbrain parts play important roles in our sensorimotor abilities (sensation and movement).
medulla: the structure at the base of the brain where it begins to widen after leaving the spinal cord; it is responsible for your heart beating and breathing
pons: a bulging area above the medulla; transfers information between the brain and spinal cord
reticular formation: an area stretched inside the medulla and pons; it is involved in attention and arousal
cerebellum: a brain area located underneath and behind the main part of the brain, it looks like a miniature brain; it is responsible for coordinating movements and helping fine-tune cognitive responses
Forebrain
Recall that the forebrain contains many structures that process sensory information, regulate our emotions, and carry out higher intellectual functions. Thus, the forebrain is the part of the brain that produces the behaviors that most clearly distinguish humans from other animals. It is no surprise, then, that the forebrain in humans is much larger than in other animals. The six major parts of the forebrain are the thalamus, hypothalamus, amygdala, hippocampus, limbic system, and cerebral cortex.
- The thalamus is a roughly oval-shaped structure above the pons, reticular formation, and midbrain. Side-view drawings of the brain make many people think that there is only one lobe of the thalamus, but in reality, there is one on each side of the brain. The function of the thalamus is to route sensory information to the correct area of the brain for additional processing. For example, light that enters through the eyes is translated to neural signals at the back of the eye (see Module 12). These neural signals are sent to the thalamus, which sends them to the part of the brain that processes vision.
- The hypothalamus is located below the thalamus (the word hypothalamus means “below thalamus”). The hypothalamus works closely with the pituitary gland, located right in front of it. Neural signals from the hypothalamus direct the pituitary gland to release chemicals called hormones into the bloodstream. These hormones travel to other parts of the body, especially to other glands, which in turn release their own hormones. As you can see in other sections throughout the book, hormones play a role in physical development, stress, sex, aggression, and other behaviors. The hypothalamus also plays an important role in motivation, including such behaviors as sex, eating and drinking, and aggression.
- The amygdala is a small almond-shaped structure (amygdalais Latin for almond) located outside and just below the thalamus (one on each side). The amygdala is probably the most important brain part for our emotions. For example, it plays a critical role in learning fear and anxiety responses. For example, because of the amygdala, if you get stung by a bee, you may feel uneasy and anxious when you see another one. The amygdala is also important for distinguishing different emotions, and for enhancing our memory of emotional episodes.
- You might recall that Module 9 described the distinction between explicit and implicit memory. Explicit memory is when you have conscious or intentional recall of some information; implicit memory does not involve conscious recall. A part of the forebrain, the hippocampus, is key to explicit, but not implicit, memory. The hippocampus is located just to the outside and a bit below the thalamus. Again, just as there are two lobes of the thalamus, you have a left and a right hippocampus. It appears that the hippocampus allows us to store new explicit memories, and it aids in the reorganization of previously stored memories to allow them to be stored for longer periods (Squire & Knowlton, 2000). So, if you remember what the function of the hippocampus is, it was the hippocampus that allowed you to do so!
- Together, the hypothalamus, amygdala, hippocampus, and a few additional parts form what is known as the limbic system, a system that appears as a ring around the thalamus (on both sides). Although the limbic system is complex and contributes to many functions, the main ones are emotion and memory. Various parts of the limbic system are involved in experiencing, expressing, and recognizing emotions. Others are important for learning, storage, and recall of information (Augustine, 2017).
- By far the most noticeable part of the human brain is the cerebral cortex, the wrinkled surface of the brain. The cortex plays a crucial role in a great many behaviors, including perception, movement, and our higher intellectual functions such as memory and reasoning. It is also better developed in humans than in other animals. Thus it deserves a bit more attention.

thalamus an oval-shaped forebrain structure that routes sensory information to other parts of the brain
hypothalamus: a forebrain area just below the thalamus; it plays a role in motivation and it controls the pituitary gland
amygdala: an almond-shaped forebrain area that is important for emotions
hippocampus: a forebrain area near the thalamus that is important for storing memories
limbic system: a group of forebrain areas that are important in emotions, among other functions
cerebral cortex: the wrinkled surface of the brain that plays important roles in perception, movement, and higher intellectual function
Cerebral Cortex
Because it is so large and so important, and its functions so diverse, the cortex is subdivided into four main sections, or lobes (really, eight when you consider that there is a version of each lobe in the left and right hemispheres): frontal lobe, parietal lobe, occipital lobe, and temporal lobe. The lobes are separated by some of the most prominent wrinkles in the cortex.
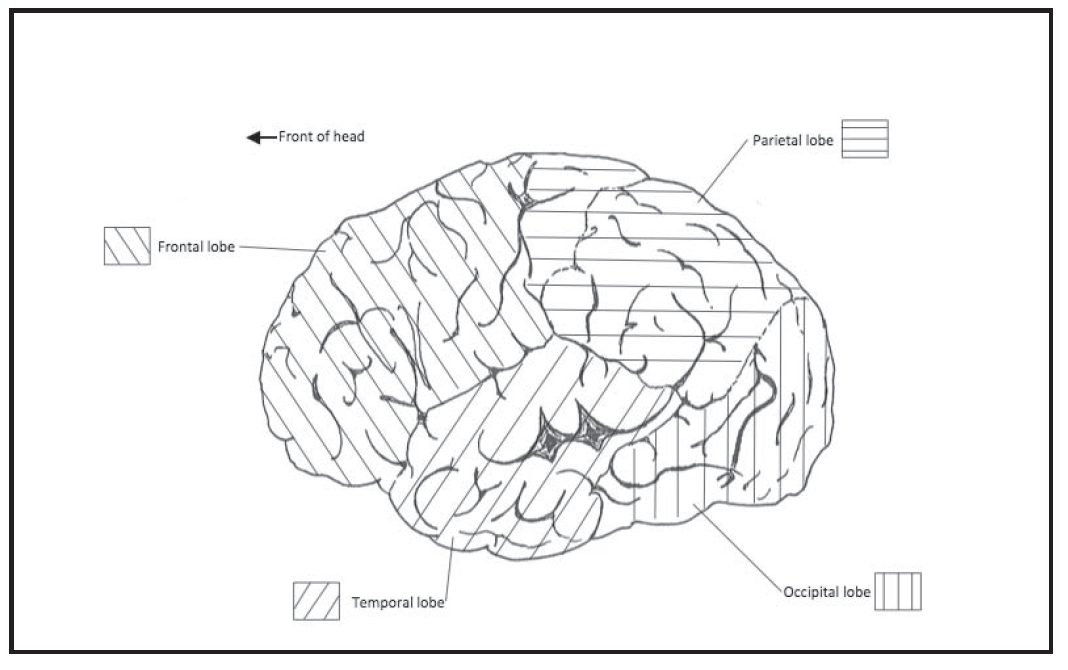

- The frontal lobe, of course, is in the front of the cortex (unfortunately, the remaining three lobes are not called topal, backal, and sidal). The frontal lobes receive and integrate sensory input that originates in all of the sense organs. They use this diverse input to help produce a great deal of complex behavior, such as judgments, planning and reasoning. The rearmost part of the frontal lobe contains the primary motor cortex, the main part of the brain that controls movements. The motor cortex in the left frontal lobe controls the right side of the body, and vice versa. The left frontal lobe contains Broca’s area, which as we have seen is involved in speech production (see a few details below in the discussion of Wernicke’s area). The front part of the frontal lobe is called the prefrontal cortex. It is the seat of many judgment and reasoning processes, and is involved in our working memory, the short-term store of information that is currently in consciousness—see Module 5 (Smith and Jonides, 1997).
- The parietal lobe is directly behind the frontal lobe. The front part of the parietal lobe is called the primary sensory cortex. It is the part of the brain that gives us our sense of touch throughout the body. The area of the parietal lobe behind the sensory cortex is important for taking in sensory information and using it to plan movements (Bizzi, 2000).
- Directly behind the parietal lobe is the occipital lobe, the area that contains the primary visual cortex. Among the lobes of the cerebral cortex, only the occipital lobe is involved with a single function, albeit a very complex one. The entire occipital lobe is devoted to visual processing.
- The temporal lobe is located on the side of the cerebral cortex, in front of the occipital lobe and below the parietal lobe. The area near the top of the temporal lobe is the primary auditory cortex, the area that processes sounds. Another important section in the temporal lobe is Wernicke’s area. For many years, this area was thought to be important for speech comprehension (and in some textbooks, it is still presented this way). Recent research has led brain researchers to conclude that it is actually important for speech production, together with Broca’s area (Binder, 2015). Indeed, Wernicke’s area is connected to Broca’s area by a large group of nerve fibers called the arcuate fasciculus. It appears that Wernicke’s area is responsible for the retrieval of speech sounds from memory, and Broca’s area is responsible for sending the commands to the primary motor cortex to move the muscles of the mouth and tongue. Speech comprehension, then, appears to reside in many other areas of the brain, including some sections in other parts of the temporal lobe and the prefrontal cortex (Binder, 2015).
arcuate fasciculus: a tract of nerve fibers connecting Broca’s area to Wernicke’s area
Broca’s area: an area in the left frontal lobe important for speech production; it works closely with Wernicke’s area in the temporal lobe
frontal lobes: the lobes in the front of the cortex that contain the prefrontal cortex and the primary motor cortex
prefrontal cortex: an area in the frontal lobes involved in judgment and reasoning, and in working memory
primary motor cortex: an area in the frontal lobes responsible for directing movement of the body
parietal lobes: the lobes of the cortex directly behind the frontal lobes; they contain the primary sensory cortex
primary sensory cortex: the section of the parietal lobes responsible for our sense of touch throughout the body
occipital lobes: the lobes of the cortex in the back; they contain the primary visual cortex
primary visual cortex: the area of the occipital lobes involved in the early processing of visual information
temporal lobes: the lobes of the cortex on the sides; they contain the primary auditory cortex
primary auditory cortex: the area of the temporal lobes responsible for the processing of sounds
Wernicke’s area: an area in the left temporal lobe important for speech production along with Broca’s area in the frontal lobe
Corpus Callosum
A short description of one additional part will complete our introduction to the brain. The corpus callosum is the main structure connecting the left and right hemispheres in the brain. This very large brain part contains axons that allow neural signals to be sent from left to right and from right to left so that the brain can function as a coordinated whole.
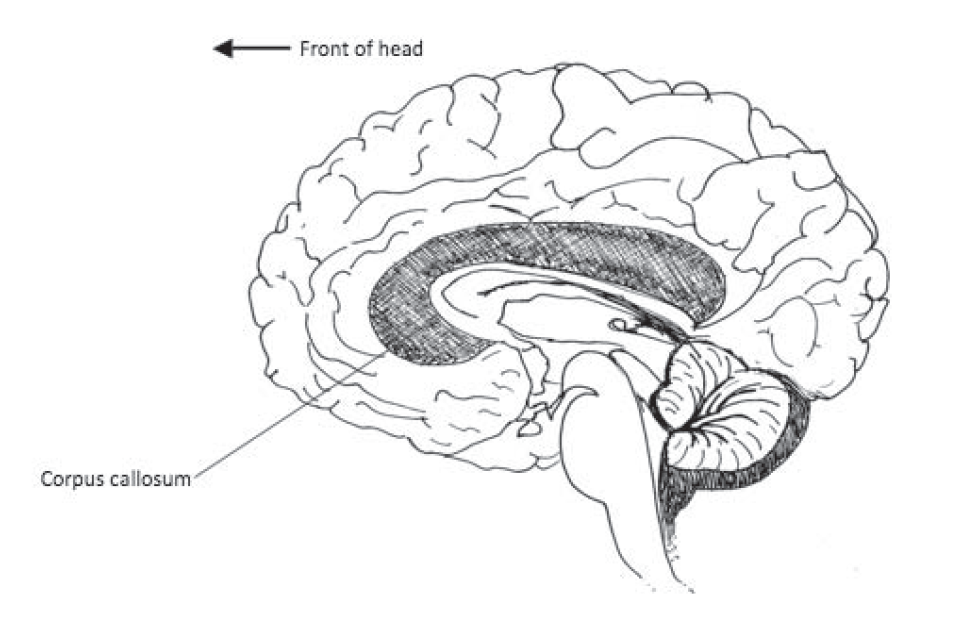
There is little doubt that the left and right hemispheres are somewhat specialized so that they typically perform different functions, and we will describe some of those differences throughout the book. For example, many verbal functions, such as speech production, are handled by the left hemisphere. It would be an oversimplification, however, to say that the left side is the language side of the brain. Consider the parts of the brain involved in listening to someone speak, for example. Much of the processing that takes place that allows us to recognize words does indeed take place in the left hemisphere. Understanding speech, however, involves much more than simply decoding words. We need to draw inferences (make conclusions about unstated information), understand subtle references and humor, read non-verbal cues and facial expressions, and so on. Many of these functions are typically handled by the right hemisphere. So, would it really be fair to say that understanding speech takes place in the left (or right) hemisphere? Because the corpus callosum is so efficient at sending information back and forth, and because so many of our behaviors are extremely complex, it is a gross oversimplification to make generalizations such as the popular notion of left-brain or right-brain dominance.
Brain Research
For the longest part of the history of brain science, the primary method for learning about how the parts of the brain relate to human behavior and mental processes was to examine case studies of patients who had suffered some kind of brain damage. For example, about 150 years ago Paul Broca discovered that many people who suffered strokes and lost the ability to speak had damage to a specific area on the left side of the brain. Broca’s area, as the part became known, was therefore assumed to be responsible for producing speech. The functions of many brain parts have been discovered this way. A second important technique has been to conduct research on laboratory animals; these researchers produced brain damage in specific areas in order to more precisely determine the function of a particular area.
Both research methodologies are quite limited, however. As you recall from the introduction to case studies in Module 2, we can never be sure if our individual case is representative of the population at large. When we are relying on individuals who are suffering from some kind of disorder or injury, we already know that there is something unusual about the people, so it becomes especially dangerous to make an automatic generalization from them. Animal research presents a similar difficulty. We must be cautious about blindly generalizing the results of animal research to humans. Many times, we find that strong relationships between brain areas and behavior in animals are much weaker in humans. On the other hand, you certainly should not automatically reject research results simply because they were based on animal research. The anatomy of nervous systems is remarkably similar across species, and neural activity, by and large, is the same across a wide variety of animals, including humans. It is definitely reasonable to draw cautious conclusions from the results of research on animals, especially if it agrees with other sources of evidence. (Recall that Module 2 contained a discussion of the specific ethical issues related to doing research with animals.)
Research that tried to assess brain activity in normal human individuals actually began about 1930 with the invention of the electroencephalogram (EEG; see Module 1 for another description). Hans Berger reported that he was able to amplify and measure electrical activity in the brain from electrodes placed on the scalp. More recently, researchers have turned to more advanced neuroimaging techniques to provide new sources of converging evidence. These techniques give us a view of brain structures and their functions, allowing researchers to examine a working human brain without being too invasive. Two common current techniques are PET and fMRI; both allow researchers to measure brain activity. PET, or positron emission tomography, allows the researcher to track glucose consumption in the brain. Glucose is the basic sugar that provides energy for body systems. Areas that are currently using a great deal of energy (because they are active) will show an increase in the consumption of glucose. In order to track the glucose, research participants are injected with a solution of radioactive glucose, which can then be detected by a radioactivity detector. The consumption of glucose can then be measured while participants complete different mental tasks. Functional magnetic resonance imaging (fMRI) measures the release of oxygen from blood cells in the brain (when brain areas use energy, one of the byproducts is oxygen, so fMRI also measures energy use in the brain). Both techniques have led to an explosion in knowledge about the functions of different parts of the brain. By the way, EEG’s are still commonly used. If you are interested to learn why a technique developed nearly 100 years ago is still a commonly used brain scanning technique, you will have to wait until Module 14.
Of course, there is much left to learn. This section has provided you with merely a brief overview of the major brain parts and their main functions. Other sections of the book provide many opportunities to expand on these ideas.
functional magnetic resonance imaging (fMRI) a brain imaging technique that measures the release of oxygen from blood cells in the brain, allowing researchers to track brain structures and their functions
positron emission tomography (PET): a brain imaging technique that allows researchers to track glucose consumption in the brain
Debrief
- Was any of the information about brain structure and function from your brain sketch or your description of a brain disorder in the Activate exercise contradicted by information from this section?
- Which separate areas of the brain do you think would have many connections between them?
11.3 Chemicals in the Brain
Activate
- Have you ever experienced “runner’s high?” If you have, how would you describe it?
- Think of the drugs and other substances, legal and illegal, that have an effect on the brain. What kind of effect do they have? Do they seem to slow down neural activity or speed it up? From what you’ve learned about the brain so far, what do you suppose is the mechanism that makes them work that way?
Caitlyn loves to run. She started running regularly when she was about 30. Prior to that, she thought that her knees were too fragile to withstand the constant pounding and could not quite understand why someone would want to run long distances. She had tried occasionally to take up the activity and might have made it 3 miles once or twice, but she honestly never did understand the appeal. The idea of running 10 miles, or even 5 for that matter, sounded more like a punishment than an activity that someone would choose. One day, though, after about a month of running 2 miles at a time a few days per week, she decided to try to run 4 miles. Her knees did not fall apart, and her lungs did not explode. It did not even hurt at all. In fact, and this is the most amazing part, she felt better at the end of the run than she had at the beginning. She had discovered “runner’s high,” and she was hooked. Nowadays, she particularly loves running in the morning, before going to work. It can put her in such a good mood that she even looks forward to coming to work on cold, snowy, dark Monday mornings in January.
What is it, this runner’s high? Is it some fictional concept that lonely runners have cooked up to trick unsuspecting non-exercisers into joining them on the streets, a cruel “misery loves company” ploy? Well, it is for real; it is not a trick. We are serious when we tell you that, for many runners, the first mile of a run hurts more than the tenth, and running really does put them in a great mood. And it looks like the whole thing is a result of neurotransmitters, those little chemicals that are released by vesicles at the axon terminal buttons of neurons and float across synapses to land on receptor sites of neighboring neurons. Not all neurotransmitters, but endorphins. Endorphins are neurotransmitters that appear to serve the function of relieving pain and elevating mood, and they are released in response to exercise. They are also released in response to injury. Caitlyn’s husband Jose once played a softball game with a broken hand that he suffered during the third inning (he batted twice with the broken hand and got two hits). He reported that it hurt a little but did not start throbbing until after the game was over. Again, Jose’s body’s natural painkillers were probably responsible.
Have you ever had surgery? If so, you were probably given a very powerful painkiller drug during your recovery, perhaps even morphine. Morphine is in the class of drugs called opiates, a class that also includes heroin, one of the most famous abused drugs. Both drugs are chemically similar to endorphins. Opiates land on the same receptor sites that endorphins stimulate and fool the sites into responding as if endorphins had been released. Thus, the pain relief and mood-enhancing properties of opiate drugs come from their mimicry of the effects of endorphins in the nervous system.
Some neuroscientists are skeptical about the endorphin explanation of runner’s high. Some deny that the concept exists (non-runners, we would guess!), but others have suggested that different neurotransmitters are responsible. For example, research has found that endocannabinoid neurotransmitters might be equally, if not more important for the feeling (Basso & Suzuki, 2017). You might recognize the word cannabinoid; these neurotransmitters are chemically similar to THC, the active drug in marijuana, both of which fit into the same receptor sites. Thus, they may produce a natural effect similar to the effects of smoking or ingesting marijuana (Dietrich & McDaniel, 2004).
If this phenomenon sounds interesting to you, you should enjoy this section. It’s about neurotransmitters and neuropsychopharmacology, the study of how drugs affect neurotransmission.
endorphins: a class of neurotransmitters that are chemically similar to opiate drugs; they function to relieve pain and elevate mood
endocannabinoids: neurotransmitters that are chemically similar to THC, the active drug in marijuana
neuropsychpharmacology: the study of how drugs affect the neural communication process
Neurotransmitters
Altogether, researchers have identified more than 50 different neurotransmitters, and observers think that there are probably hundreds (Moini & Piran, 2020; Sapolsky, 2004). Most neurons release only one kind of neurotransmitter, but because they receive input from many different neurons, they receive many different neurotransmitters. As we are sure you can guess, with so many neurotransmitters, each one a key for a specific receptor site, the neural communication process can get very complicated. To further complicate matters, each individual neurotransmitter may have many different types of receptors that it binds to, so the keys can open a few different locks each. Consider one of the most famous neurotransmitters, serotonin, which appears to be involved in mood, aggression, appetite, cognition, vomiting, motor function, perception, sex, and sleep, along with some additional processes (Aghajanian & Sanders-Bush, 2002). There are at least 14 different subtypes of serotonin receptors throughout the brain, which helps explain how it can be related to so many different functions.
When you contemplate this kind of complexity, you can begin to see how the astonishing hypothesis—that idea that everything you think and feel can be traced to electrochemical activity in your brain—could be true. Think about it: if there are 300 neurotransmitters, each with an average of 5 different kinds of receptor sites that it will bind to, roughly 1,500 different types of synapses are required.
Let us briefly mention some of the most important neurotransmitters and a few of the functions with which they are involved. Depending on where in the nervous system the neurotransmitters appear (and the type of receptor sites), they may contribute to many different functions.
Remember from section 11.1 that neurotransmitters may be excitatory or inhibitory. The most common excitatory neurotransmitter in the brain is glutamate, and the most common inhibitory neurotransmitter is gamma-aminobutyric acid, abbreviated GABA (Nestler & Duman, 2002). The release of glutamate by axons in the reticular formation leads to arousal. The release of glutamate in the hippocampus and probably other brain regions appears to be related to the brain’s ability to change permanently as a consequence of experience—that is, to learn (Malenka & Nicoll, 1999). Because they are producing changes, you can easily see how these two effects could be considered excitatory (although it is not always this obvious). The other side of the coin is the inhibitory neurotransmitter GABA. When released by neurons in the amygdala, GABA reduces anxiety. GABA released in sensory areas of the brain may help with our ability to integrate input from different senses into a coherent whole (King & Schnupp, 2000). For example, if a friend is talking to you, your brain has to combine the visual and auditory input—processed by different brain areas—into a single experience.
Acetylcholine is a very common neurotransmitter in the peripheral nervous system. It can be excitatory or inhibitory. In its main excitatory role, acetylcholine is released by motor neurons and stimulates the muscle cells that produce movement. It is also the main neurotransmitter used by the parasympathetic nervous system, the part of the autonomic nervous system that calms that body down.
Norepinephrine and dopamine are chemically very similar to each other; norepinephrine is synthesized from dopamine, in fact (Byrne, 1997). Dopamine is usually an excitatory neurotransmitter; its release in the midbrain and some areas of the forebrain is related to reward, or pleasure (Drevets et al., 2001; Wise, 2004). Dopamine in the prefrontal cortex is related to working memory (Goldman-Rakic, et al., 2000). Norepinephrine, which can be excitatory or inhibitory, is the main neurotransmitter used by the sympathetic nervous system, which is what controls the “fight or flight” response. Norepinephrine also appears to be related to mood.
A few of these neurotransmitters are part of the discussion in other sections of the book. For now, here is a summary of these important neurotransmitters and some examples of their effects:
Neurotransmitter | Excitatory or Inhibitory | Important Example Effects |
Acetylcholine | Excitatory at muscle sites; otherwise excitatory or inhibitory | Produces movement and parasympathetic activity |
Dopamine | Usually excitatory | Enhances pleasure and working memory |
GABA | Inhibitory | Reduces anxiety, integrates sensory input |
Glutamate | Excitatory | Increases arousal and learning |
Norephinephrine | Can be excitatory or inhibitory | Affects mood and sympathetic nervous system activity |
Serotonin | Usually inhibitory | Affects mood, aggression, sexual response, and many other behaviors |
acetylcholine: a common neurotransmitter in the peripheral nervous system
astonishing hypothesis: that idea that everything you think and feel can be traced to electrochemical activity in your brain
dopamine: a neurotransmitter that is released in the midbrain and some areas of the forebrain that is related to reward
gamma-aminobutyric acid (GABA): the most common inhibitory neurotransmitter
glutamate: the most common excitatory neurotransmitter in the brain
norepinephrine: the main neurotransmitter used by the sympathetic nervous system
serotonin: a neurotransmitter that appears to be involved in mood, aggression, appetite, cognition, vomiting, motor function, perception, sex, and sleep, and additional processes
Neuropsychopharmacology
Long before we had any idea about neurons and neurotransmitters, human beings sought to influence the levels of neurotransmitters. Natural remedies, consciousness-altering substances, even some primitive weapons were used by people because the effects on neurotransmission were desirable (for example, pleasurable or, in the case of weapons, deadly). For example, people in South America have eaten the extract of coca leaves for centuries. Today, that extract is processed into cocaine. Curare is a substance that South American native tribes have used to paralyze and kill prey. Both curare and cocaine have their effects on neurotransmission (curare on acetyocholine, cocaine on dopamine).
Today we call substances that have some kind of psychological effect neuropsychopharmacological drugs; they work by influencing the neural transmission process in some way. They include clinical drugs that are used to treat psychological disorders, clinical drugs used to treat other disorders that have psychological side effects, and recreational and abused drugs, such as nicotine, alcohol, or cocaine.
Currently, biopsychologists and medical doctors divide neuropsychopharmacological drugs into two categories; they can be classified by whether they increase or decrease the effects of a neurotransmitter. Those that increase the effects of neurotransmitters are called agonists; those that decrease the effects are called antagonists. In addition, neuropsychopharmacological drugs work on both inhibitory transmitters and excitatory transmitters. This table will help you understand how these two dimensions interact to create four basic types of neuropsychopharmacological drugs.
Effect on Brain Activity | Inhibitory Neurotransmitter | Excitatory Neurotransmitter |
Increase activity (Agonist) | Example: Valium | Example: Cocaine |
Decrease activity (Antagonist) | Example: Absinthe | Example: Antihistamines |
- Agonist for inhibitory neurotransmitter: Valium (chemical name: diazepam) increases the activity of the neurotransmitter GABA, which you will recall decreases anxiety when it is released in the amygdala. Hence, Valium acts as an antianxiety drug. Because GABA also has other functions, GABA agonists, such as Valium, have other effects as well. For example, Valium can cause sleepiness and memory impairment (Rudolph et al., 1999). These unintended effects are called side effects, but they are simply the natural effects of influencing the activity of a neurotransmitter on untargeted areas of the nervous system.
- Agonist for excitatory neurotransmitter: Cocaine increases the activity of dopamine. Recall that dopamine release is related to pleasure, so cocaine has quite direct effects of increasing pleasurable feelings.
- Antagonist for excitatory neurotransmitter: Many people take antihistamines to treat allergies. They work by inhibiting the activity of the excitatory neurotransmitter called histamine; histamine from the hypothalamus causes arousal. Antihistamines therefore may lead to sleepiness. (The curare used to paralyze and kill prey is also an antagonist for an excitatory neurotransmitter, acetylcholine.)
- Antagonist for inhibitory neurotransmitter: Antagonists of inhibitory neurotransmitters are not common. One fascinating example is oil of wormwood, the special ingredient of the liqueur absinthe. Absinthe was rumored to be a powerful hallucinogen (in addition to being a very potent liqueur); it was consumed by many creative people in the 19th and early 20th centuries. Mary Shelley was purported to be under the influence of absinthe when she wrote the novel Frankenstein. Although the actual hallucinogenic properties of oil of wormwood are not known, it is clear that the substance is an antagonist for GABA (Olsen, 2002), which helps to control sensory input.
Although this two-by-two organization is fairly simple, it gets complicated very quickly when you realize that agonists and antagonists can have their effects by different mechanisms. For example, an antagonist might work by blocking the receptor sites, by blocking the presynaptic membrane so that less of the neurotransmitter is released, or by chemically breaking down the neurotransmitter while it is floating across the synapses. An agonist might work by causing more of a neurotransmitter to be released from a synapse, by landing on a receptor site and “fooling” it into opening up, or by keeping neurotransmitters in a synapse for a longer time.
In order to understand one of the most important agonistic mechanisms—keeping neurotransmitters in the synapse—you need to know one more detail. Most neurotransmitters—for example, serotonin and dopamine—are released from the receptor sites and reabsorbed by axon terminals; the process is called reuptake. An agonist often works by interfering with the reuptake process. For example, cocaine gets its agonistic effect on dopamine this way; it encourages neural activity in part by keeping this excitatory neurotransmitter in the synapses. Similarly, Prozac and Zoloft are antidepressants that have agonistic effects on serotonin by inhibiting its reuptake. These antidepressants are called (accurately but not gracefully) selective serotonin reuptake inhibitors (SSRIs).
agonist: a drug that increases the activity of a type of neurotransmitter
antagonist: a drug that decreases the activity of a type of neurotransmitter
neuropsychopharmacological drugs: drugs that work by influencing the neural transmission process in some way
reuptake: the process of reabsorption of neurotransmitters into axon terminal bulbs after their use in a synapse
Debrief
- Can you describe any other kinds of experiences that seem similar to runner’s high?
- Review your list of common drugs that have psychological effects. It may include, along with other drugs you may have listed, alcohol, nicotine, caffeine, and marijuana. Which of these drugs do you now think is more likely to be an agonist or an antagonist?