33. Nuclear physics and radioactivity
Summary
The atomic nucleus
Recall that the results of Ernest Rutherford’s gold foil experiment in 1911 led to the discovery of the atomic nucleus. The nucleus was found to have a positive charge, contain almost all the mass of the atom, and have a diameter that was about the diameter of the atom itself (for very light atoms) and about
(for very heavy atoms). With this discovery, the atom came to be viewed as a very tiny positively charged nucleus with electrons orbiting around that nucleus in a planetary fashion (in that electrons move around the nucleus, similar to how planets move around a star) as it is most commonly depicted in textbooks. This planetary model is depicted in Figure 33.1. However, such diagrams, such as the one shown in Figure 33.1, are not to scale. To put the scale of an atom in perspective, if the atomic nucleus was the size of a baseball, then the atom itself would be about 8 km (or about 5 miles) in diameter. In other words, atoms are mostly empty space!
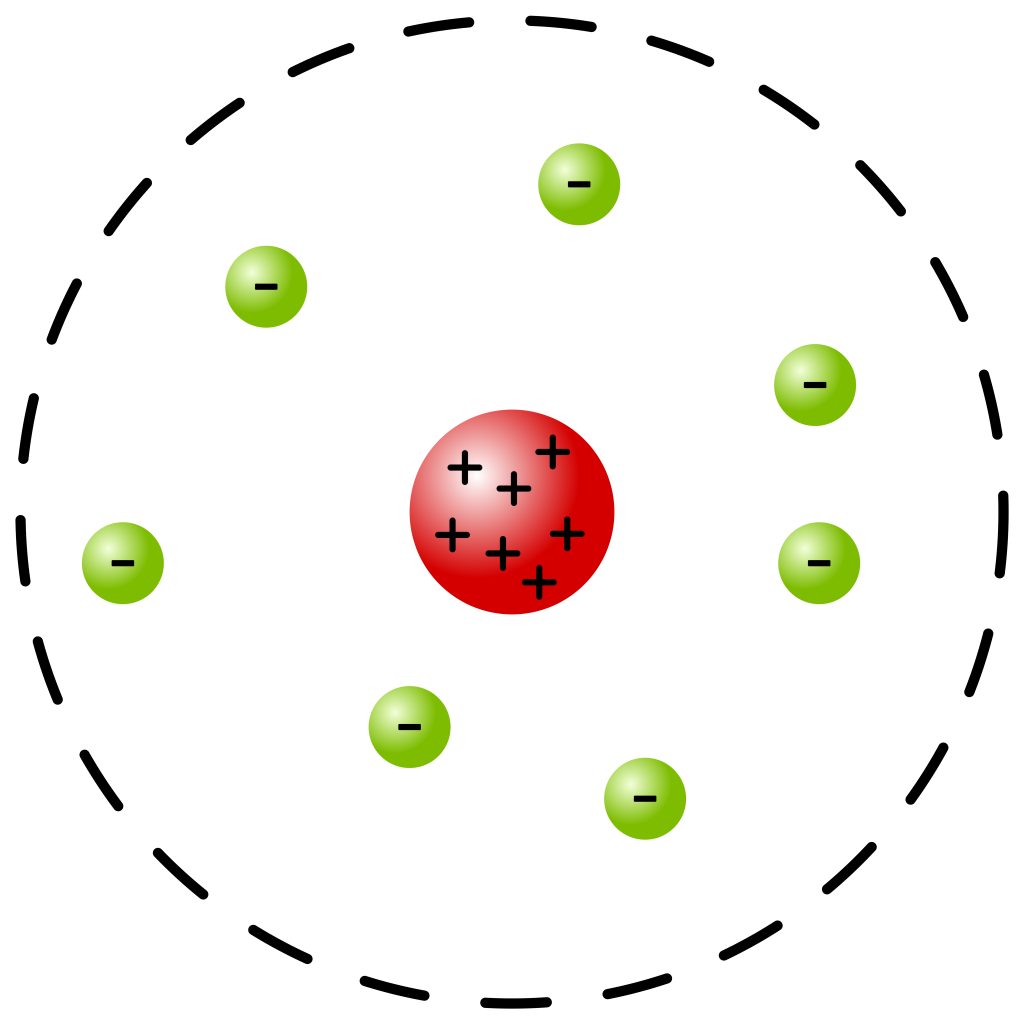
Subsequent experimentation by Rutherford later showed that hydrogen nuclei were present in all atomic nuclei. As a result, Rutherford is also credited with the discovery of the (positively charged) proton in 1917. Although we now know that the atomic nucleus is composed of protons and neutrons, it was not until 1932 that the (electrically neutral) neutron was discovered. So the planetary model predicted by Rutherford was modified again to include an atomic nucleus, composed of protons and neutrons, which was surrounded by electrons. Collectively, protons and neutrons are referred to as nucleons.
If there are positively charged protons in the nucleus, the question arises, what keeps the protons clumped together? Recall that like charges repel. Positive charges repel other positive charges. Why don’t nuclei repel themselves apart due to the electrostatic repulsion among the protons? The answer requires that there be another kind of interaction whose attractive strength overpowers the electrostatic repulsion of the protons. This new force is called the strong nuclear force. The nuclear strong force is the strongest (hence its name) of the four known fundamental forces of nature. (The other three are electromagnetism, the weak nuclear force, and gravity.) The table below shows the basic properties of each of the four fundamental forces.
force |
properties
|
relative strength*
|
range (m) |
strong nuclear |
binds quarks together and binds nucleons together (attractive)** |
1 |
|
electromagnetism |
holds atoms together and binds atoms into molecules (attractive and repulsive) |
~0.01 |
infinite |
weak nuclear |
responsible for particle decay and radioactivity (neither attractive nor repulsive) |
~ |
|
gravity |
holds large-scale structures (solar system, galaxies, etc.) together (attractive) |
~ |
infinite |
* Compared to the strong nuclear force.
** There are instances where the strong force can be repulsive, but the discussion of the details is beyond the scope of this textbook.
Other than the fact that the strong force is literally holding all of our constituent atomic nuclei together, we do not directly experience the strong force in our daily lives. It is not something that we can feel like gravity, or experience like electromagnetism. Particles that interact through either electromagnetism or gravity can be any distance apart. On the other hand, the strong nuclear force is extremely short-ranged and only attracts the nearest neighbor nucleons.
In small nuclei, the strong nuclear force will easily keep the protons together despite their electrical repulsion. The presence of the electrically neutral neutrons in atoms also helps with atomic stability by creating a buffer between the repeling protons. If an atom has lots of protons, the size of the atomic nucleus becomes quite large. At a certain point, the size scale of an atomic nucleus may be larger than the length scale that the strong force can operate. These atomic nuclei are unstable, and undergo radioactive decay. Elements with more protons than lead (elements with an atomic number greater than 82) all undergo spontaneous decay.
Consider a crude analogy: imagine stacking fluffy pillows one on top of the other into a tower. Each added pillow increases the potential energy of the system. It is easy to stack the first few pillows. However, as the stack gets taller, it becomes harder to stack the next pillow due to the structural instability of the tower. The support forces eventually give way to gravity: the tower tumbles and releases the potential energy as the pillows fall.
As discussed previously in this textbook, isotopes are elements that have the same number of protons but different numbers of neutrons. Many elements have multiple isotopes. Some of those isotopes may be stable, and some may be unstable. Interestingly, neutrons themselves are intrinsically unstable and decay spontaneously. It is only in the environment of the nucleus that the strong force of neighboring nucleons keeps the neutrons stable. However, a nucleus with too many neutrons will be unstable and undergo decay. When the strong force is no longer able to hold the mutually repulsive protons and the neutrons together, the atomic nucleus decays with the release of energy. This nuclear decay is referred to as radioactivity.
Radioactivity
Just a few months after the discovery of x-rays, Henri Becquerel serendipitously discovered a new form of radiation when uranium salts left in a drawer caused a nearby photographic plate to become exposed (this photographic plate is shown in Figure 33.2). Unlike other high-energy particles such as x-rays (which are also capable of exposing a photographic plate in this manner, and which are purposefully generated using a cathode-ray tube), this new radiation occurred spontaneously. Furthermore, scientists performed experiments and learned that whatever was emitted by the uranium could not be mere x-rays, because the particles emitted by the uranium could be deflected by electric and magnetic fields. This means whatever the uranium was spitting out contained an electric charge. This was the discovery of spontaneous radioactivity. It was subsequently found that there are three primary types of radiation. These three types were named after the first three letters of the Greek alphabet: alpha (), beta (
), and gamma (
). These will be discussed in turn starting in the next section.
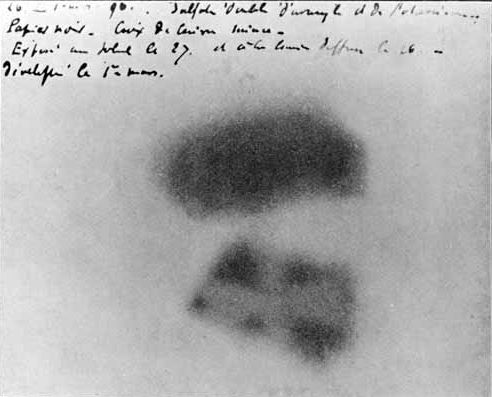
It should be pointed out that x-rays are an atomic phenomenon resulting from electron deexcitations from higher shell electrons dropping into lower shells of the electron cloud surrounding the nucleus. However, radioactivity is a nuclear phenomenon resulting from drastic changes taking place within the nucleus of an atom. It is also interesting to note that these nuclear transitions were discovered more than a decade before the nucleus itself was discovered!
The discovery of radioactivity initially unleashed a lot of new knowledge about atoms and isotopes. In particular, the study of radioactivity and radioactive elements was expanded by the work of Marie Curie, who received two Nobel Prizes for her work in the discovery of radioactivity and isolation of the elements radium and polonium.
Before we learned about the negative effects of radiation exposure, the discovery of radioactivity also led to an enthusiasm for radioactive products (many of which were pseudoscientific), such as radium-infused water (shown in Figure 33.3), that people would consume, not realizing that radioactivity is dangerous and can cause cancer and other health hazards in high dosages.
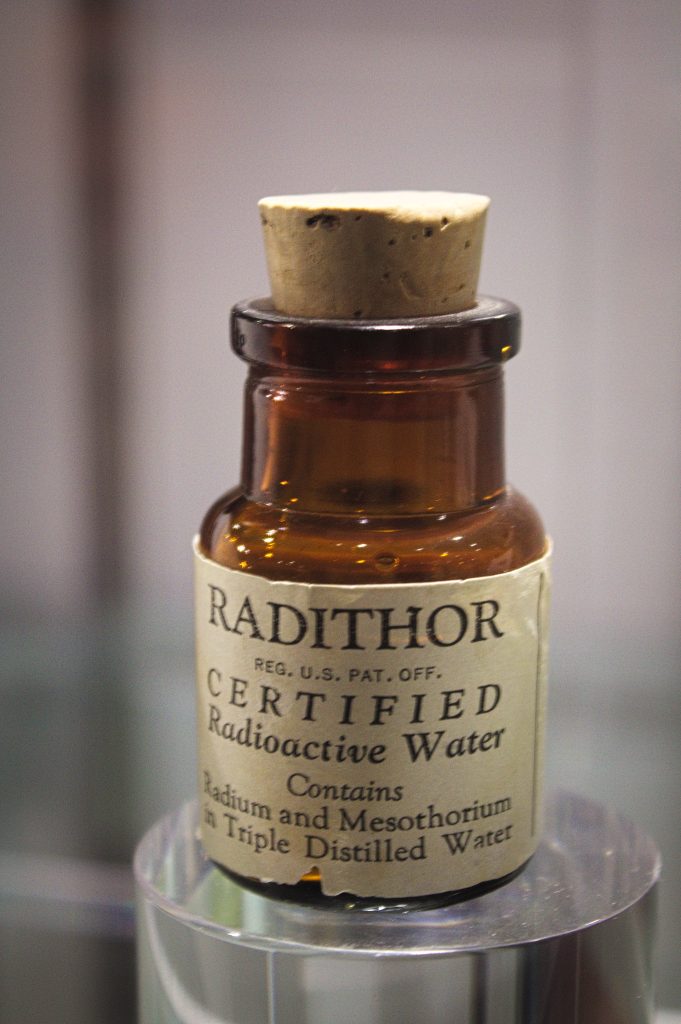
Not just pseudoscientific products contained radioactivity. Some commercial products were designed with transuranic elements, even before the fission process was fully understood. Uranium glass is a type of glass that uses uranium to achieve a brilliant green coloration. Some red/orange glazes used in ceramics and tiles used uranium to obtain the coloring. Fiesta brand dinnerware manufactured in the middle of the 20th century contained uranium, and is known to be radioactive. A radioactive Fiesta brand dinner plate is shown in Figure 33.4.
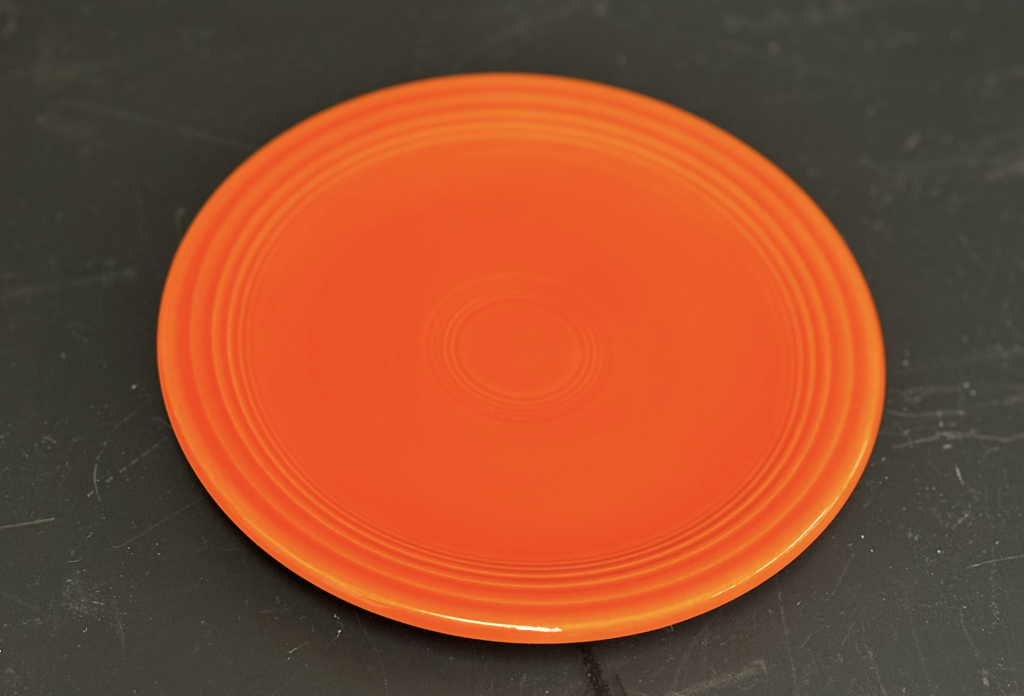
In the video below, Dr. Fazzini and Dr. Pasquale use a Geiger counter to measure the decay particles emitted by the uranium in the ceramic glaze of a Fiesta brand plate. When a lead brick is placed between the plate and the Geiger counter, the reading on the Geiger counter goes back to regular background levels of radiation.
Fortunately, our knowledge of radioactivity and radiation is much more thorough now that we’ve had more than 100 years to learn more about it.
As we’ll discuss to a small degree in this chapter, there are many beneficial uses of radioactivity in our lives. Medical sources of radiation can be used to diagnose and treat otherwise lethal conditions such as cancer. Carbon dating is used to determine the age of organic artifacts back to about 50,000 years. Uranium dating can be used to determine the age of ancient rocks that are billions of years old. Scientists use radioactivity to create new atoms and isotopes. Smoke detectors use a radioactive source to detect the presence of smoke. The interior of the Earth remains hot due to the energy released in the radioactive decay of unstable nuclei deep in the Earth (this is the source of geothermal energy).
Alpha decay
Alpha decay is a radioactive decay process wherein a nucleus emits a particle known as an alpha particle. This particle was found to have a positive electric charge and could be blocked using just a piece of paper. It was not until 1909 that Rutherford discovered that an alpha particle was a helium nucleus. It is now known that a helium nucleus consists of two protons and two neutrons. The strong force happens to hold these four nucleons into a very tightly bound entity. When alpha decay occurs, it is generally easier for the unstable nucleus to eject an entire helium nucleus rather than a single proton. (Although proton emission can occur, it is extremely rare.) The process of alpha decay is depicted in Figure 33.5.
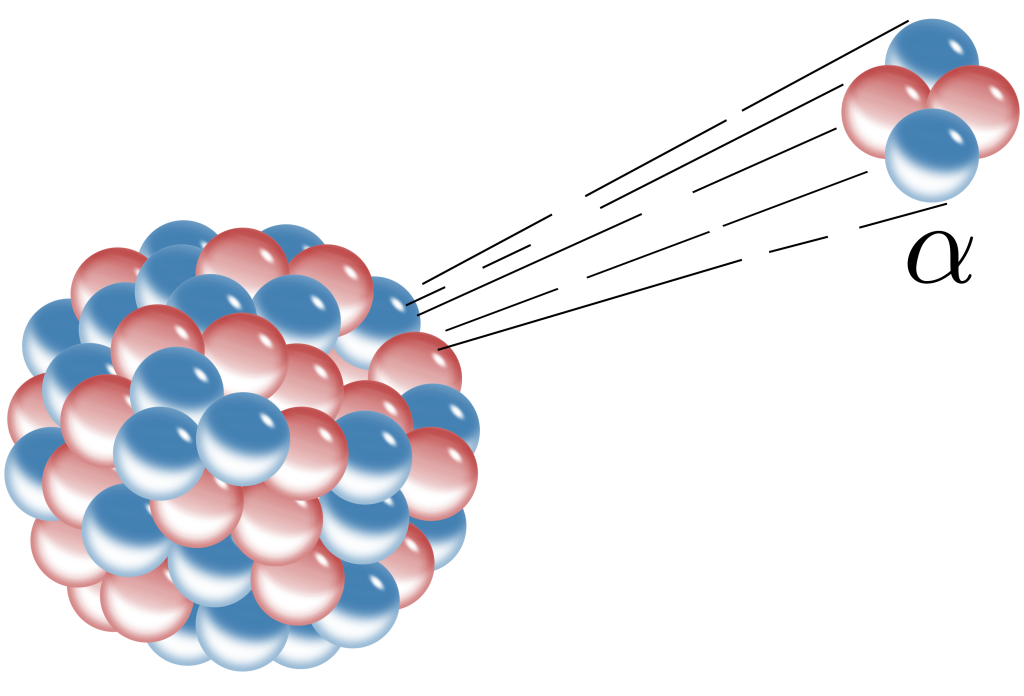
During the alpha decay process (and, as will be discussed, all of the radioactive decay processes), the total charge and total energy of all particles is conserved, as is the total number of nucleons in all of the particles.
The composition of alpha particles was determined using the magnetic deflecting force described earlier in this textbook. This deflection is shown in Figure 33.6.
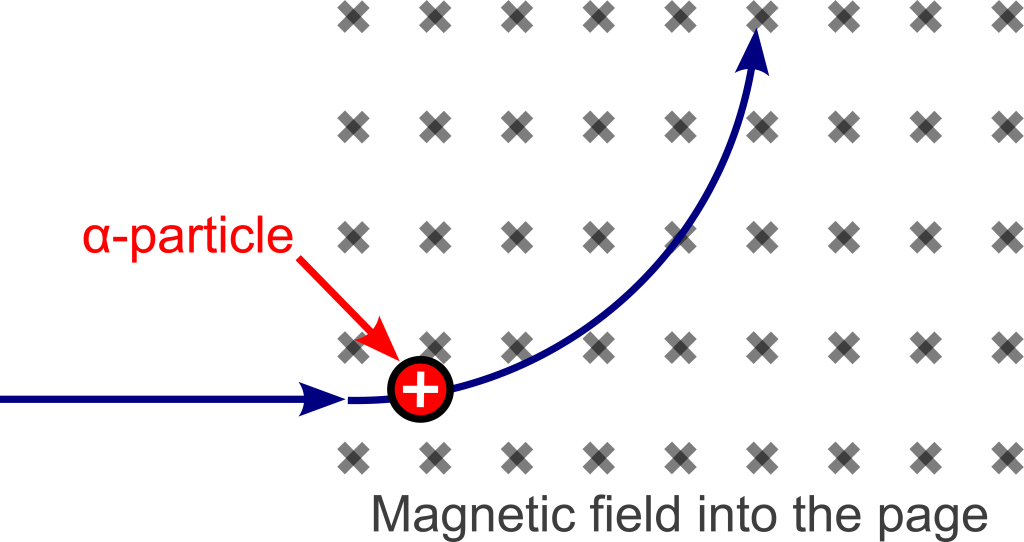
The amount of deflection that a charged particle undergoes gives information about its charge and mass. Because of inertia, a heavy particle will deflect less than a light particle. The direction of deflection has to do with the sign of its electric charge. In 1909, American physicist Robert Millikan measured the elementary charge of the electron. Once the elementary charge was known, the masses of the deflected particles could be determined. (This is because the particle, traveling in a circular path, requires a centripetal force, contributed by the magnetic deflection force. Knowledge of the elementary charge is necessary to calculate the mass of the charged particle once the radius of the circular path is known.) The deflection direction of alpha particles (which can be determined by the right-hand rule) is consistent with that of a positive charge.
Because alpha particles are so massive, they are very likely to lose energy when coming into contact with any other atoms. So while they are dangerous, they can be blocked by anything more massive than, say, a sheet of paper. It’s therefore relatively easy to shield against alpha particle exposure.
An example of alpha decay occurs when uranium-238 emits an alpha particle and becomes thorium-234:
The atomic mass number is conserved. There are 238 nucleons in the original atom of uranium. The final atom of thorium has 234 nucleons, and the other 4 are present in the alpha particle. Atomic number (charge) is also conserved. The original uranium atom has 92 protons. The final thorium atom has 90 protons, and the alpha particle has the remaining 2.
In general, the alpha decay of any isotope is depicted by the equation:
where and
are the atomic mass number and atomic number of the unstable nucleus, respectively. The letter
represents the nuclides (nuclei of a particular isotope) before the decay, while
represents the nuclides after the decay. The nuclide on the left side is called the parent nucleus while the one on the right side is called the daughter nucleus.
A cloud chamber is a device that can be used to track the byproducts of radioactive decay. In the video below, lead-210 that is placed in the center of the chamber undergoes alpha decay, which generates condensation in an alcohol vapor inside the chamber and can be visualized as trails of clouds emanating from the source.
Beta decay
Beta decay is a radioactive decay process wherein a nucleus emits an electron. Before the identity of this particle was known, experiments with magnetic fields showed that this byproduct of beta decay contained a negative charge, had the same mass as an electron, and was more penetrating than alpha particles. This may seem peculiar since we do not find electrons in the nucleus. What is going on here?
Beta decay is a process whereby a neutron in a parent nucleus converts to a proton, and while doing so emits an electron and another subatomic particle now known as an anti-neutrino. This decay is depicted in Figure 33.7. During this process, the total charge and energy of all particles is conserved, as is the total number of nucleons in all of the particles. The proton remains in the daughter nucleus after the electron is shot out. This particular process is referred to as decay due to the emission of the negatively charged electron from the nucleus.
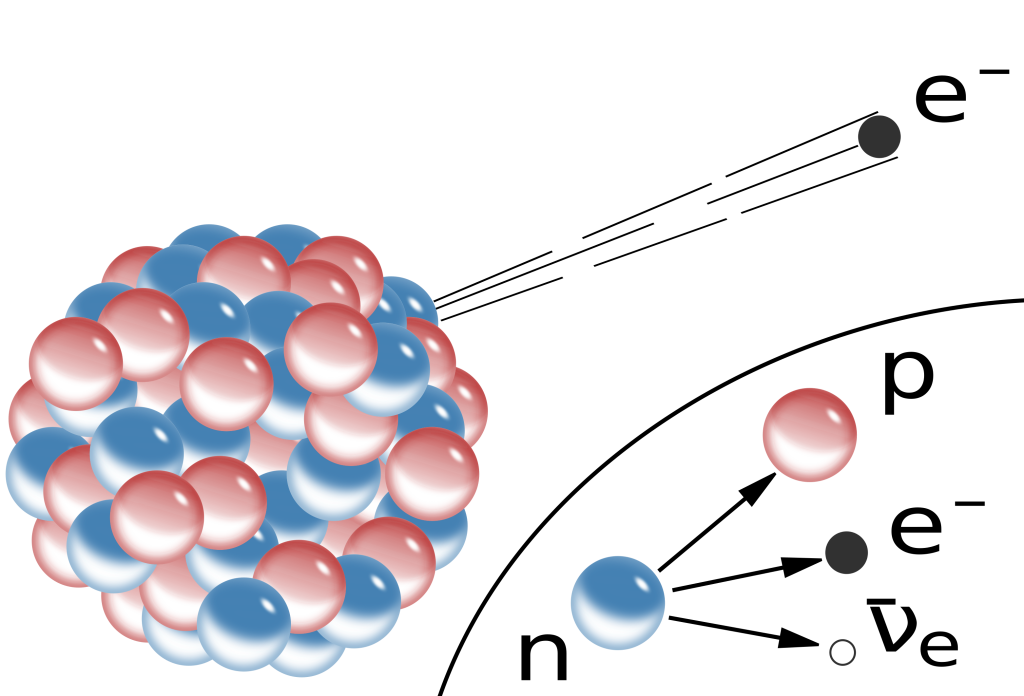
Because they are smaller than alpha particles, beta particles are more penetrating than alpha radiation. Therefore, they require thicker shielding to prevent exposure. However, it is possible to protect against beta particles by using a few millimeters of aluminum, for example.
An element that undergoes decay is thorium-234. An atom of thorium-234 will emit an electron and become protactinium-234:
Note that the atomic mass number is conserved. There are 234 nucleons in the original atom of thorium, and 234 nucleons in the final atom of protactinium. The atomic number (charge) is also conserved. There are 90 protons in thorium. There are 91 protons in protactinium, balanced by one electron.
In general, decay can be summarized by the equation :
(The notation associated with the electron is to remind you that the electron consists of zero nucleons and has an electric charge of -1. Also, the anti-neutrino is omitted from the equation for simplicity.) After this process, the number of protons has increased by one at the expense of the neutron that decayed, but the total number of nucleons is unchanged.
In 1934, an “opposite” type of beta decay was observed by Irene and Frederic Joliot-Curie (a husband and wife who were the daughter and son-in-law of Marie and Pierre Curie). In this decay, a proton in a parent nucleus converts to a neutron that remains in the daughter nucleus, while a positron and a neutrino are ejected from the daughter nucleus. As discussed earlier in this textbook, a positron has the same mass as an electron but has a positive electric charge. Consequently, this decay process is referred to as decay or positron emission.
Gamma decay
An unstable nucleus that has just undergone alpha or beta decay may be left in an excited state. Just as there are electron energy levels in which electrons can become excited and decay to a lower energy level, protons and neutrons within the nucleus are also arranged in energy levels. A nucleus in an excited state can decay to a lower energy level with the emission of a photon. This nuclear decay process is known as gamma decay. And just as an atom emits a photon when an electron drops to a lower orbital energy level, a nucleus can deexcite and emit gamma radiation. As the energy levels in the nucleus are thousands of times more energetic than the x-rays emitted from atomic electron transitions, gamma radiation is electromagnetic radiation that is thousands of times more energetic than x-rays. In short, gamma radiation is simply a very high-energy photon and is a form of ionizing radiation. This process is depicted in Figure 33.8.
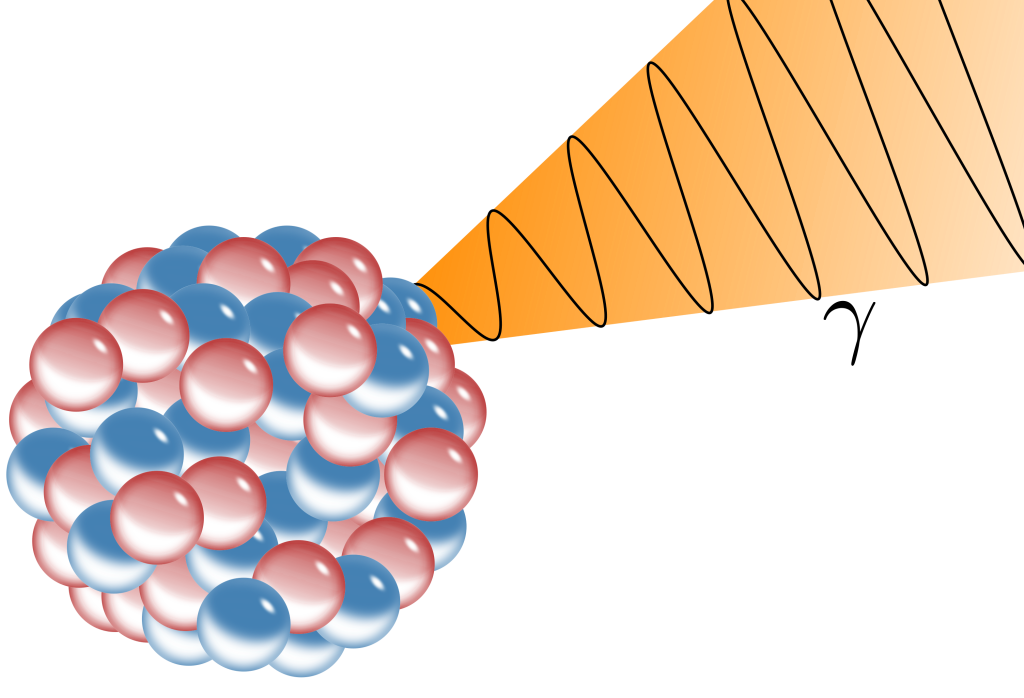
Because gamma radiation is unaffected by the magnetic deflection force, it was shown to have no charge. Because it exhibits characteristics of electromagnetic waves (such as traveling at the speed of light in free space), it was determined to be part of the electromagnetic spectrum.
Gamma radiation can be summarized by the equation:
where the asterisk denotes that the unstable nucleus is in an excited state. Note that after the gamma decay, the daughter nucleus is the same isotope since neither the atomic mass number nor the atomic number is unchanged after the decay.
Gamma radiation is very hazardous and also more difficult to shield against than alpha or beta radiation. Gamma rays can be blocked with thick blocks of lead, concrete, or even large amounts of water.
Other decay modes
In addition to the three basic radioactive decays (four if you count decay), there are other decay mechanisms that can occur. Four additional processes are briefly listed below (although there are other extremely rare modes that are not listed):
- proton emission (in which a nucleus ejects a single proton), which is an extremely rare process,
- neutron emission (which occurs in some neutron-rich isotopes as a result of a repulsive effect of the strong nuclear force),
- electron capture (in which a nuclear proton and an atomic electron merge to form a nuclear neutron with the emission of a neutrino, also called reverse beta decay), and
- neutron capture (in which a nucleus absorbs a neutron).
All of the decay processes discussed are summarized in the table below.
process |
equation |
example |
alpha decay |
|
|
beta-minus decay |
|
|
beta-plus decay (positron emission) |
|
|
gamma decay |
|
|
proton emission |
|
|
neutron emission |
|
|
electron capture (reverse beta decay) |
|
|
neutron capture |
|
|
It should be noted that the three beta decay modes described in the table above are mediated by the weak nuclear force. Because the weak nuclear force is neither attractive nor repulsive (in that it does not accelerate particles), a better term is the weak nuclear interaction. The weak interaction is responsible for changing quarks from one type to another. It is the changing of the quarks’ types that converts neutrons into protons and vice versa. Further discussion of this topic is beyond the scope of this textbook.
Half-life
The decay process of unstable elements is characterized by something known as the half-life. This half-life is a description of the amount of time it takes, on average, for half of a particular isotope to decay. Every time a half-life elapses, half of the sample (on average) will have undergone the radioactive decay process, leaving half in the original unstable state. Because the subsequent decay occurs with half of the remaining sample, the percentage of the sample that has not undergone the decay process yet will decrease exponentially over time. This is depicted in Figure 33.9.
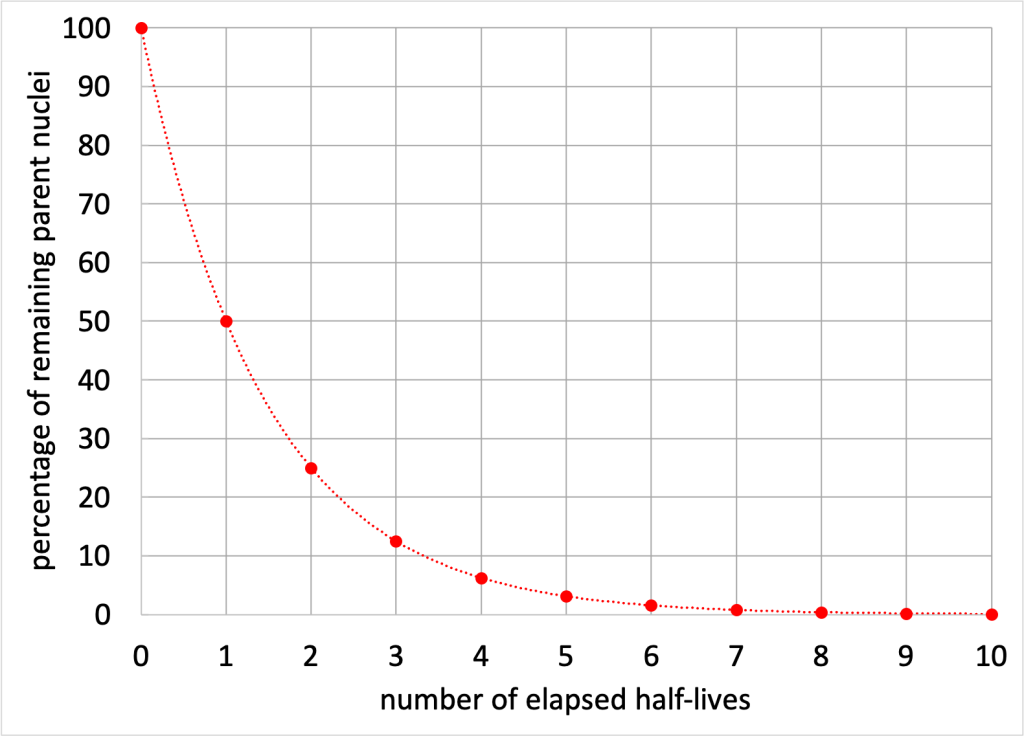
A decay process is probabilistic (similar to rolling dice or flipping coins). For example, when rolling a fair single 6-sided die, it cannot be said with any certainty that the next roll will result in any particular number (such as the number 1, for example). What can be said is that there is a one-in-six chance that the next roll will result in any particular number. Even if a die has been rolled a hundred times without getting a 1 (an unlikely but not impossible occurrence), there is still only a one-in-six chance that the next roll comes up 1.
As another example, tossing a coin 100 times will likely result in roughly equal amounts of heads and tails outcomes. However, during this experiment, it is possible that multiple or even many subsequent coin tosses may result in all “heads-up” or all “tails-up.” Each coin toss is an independent event with a 50% chance of obtaining a particular outcome.
Similarly, any particular nucleus of an unstable isotope may decay in a timeframe that is either longer or shorter than the known half-life. In other words, there is no way to predict whether a particular nucleus will decay within a certain time. All that can be said is that within a certain time frame, a certain fraction of unstable parent nuclei will decay (on average).
Every isotope has its own half-life. Xenon-124 has one of the longest known half-lives of years, much, much, much older than the known age of the universe! Scientists have detected the decay of xenon-124, even though it is an extremely rare occurrence.
On the other end of the spectrum, a synthetic isotope of hydrogen, hydrogen-5, has the shortest known half-life of seconds.
Because the half-life defines the amount of time it takes for half of an unstable isotope to decay, how long would it take 100% of an unstable isotope to decay? Consider an isotope with a mass of 100 grams and half-life of one day. After one day, approximately 50% (50 grams) of the sample will have decayed. After two days, approximately 50% of the remaining unstable sample, or 25 grams, will decay. After three days, an additional 12.5 grams will decay. This means that after three days, approximately 87.5% of the sample will have decayed.
After 7 days, one week, about 99.2% of the sample will have decayed. After two weeks, about 99.99% of the sample will have decayed. When does that value become 100%? The answer is that the decay process is asymptotic. We see that it approaches 100% over a long period of time, but the answer to the question of “when does the very last atom of the isotope decay” is completely probabilistic and cannot be definitively answered.
Unstable isotopes with long half-lives that are present in nuclear waste or contamination can be particularly dangerous. This is because the long half-life indicates that a lot of time will have to pass before the isotope decays to a safe level. Even when the isotope does decay, it may decay to a second unstable isotope. It’s possible that it will take days, years, decades, centuries, millennia, or longer for half of some radionuclides to decay to a stable isotope.
Carbon dating
Carbon dating is used to determine how old organic archeological samples are. All organic objects contain carbon. Living plants and animals will naturally replenish this carbon, which includes carbon-12, a stable isotope, and carbon-14, an unstable isotope that undergoes beta decay. After death, the carbon-14 will no longer be replenished and will undergo beta decay with a half-life of 5,730 years. This means that the ratio of carbon-14 relative to carbon-12 in an artifact can be used to calculate its age with reasonable accuracy to about 50,000 years.
Radiation dosage and exposure
The amount of radioactivity that humans encounter can be characterized by the energy we absorb from that radioactivity. This is known as rad: radiation absorbed dose. One rad is equivalent to 0.01 joules per kilogram of tissue.
However, not all radiation is equally dangerous. The unit of rem, roentgen equivalent man, attempts to quantify the dosage of radiation based on the damage it causes to humans. Alpha radiation, for example, may only emit 1 rad, but has a health effect of 10 rems. Beta radiation of 1 rad would have a health effect of 1 rem.
A lethal dose of radiation for a human starts at about 500 rem distributed over the entire body. Such an exposure causes death to 50% of the population within 30 days. Localized dosages of equivalent amounts of radiation may be survivable.
While the concept of radioactivity sounds scary, the truth is that we are surrounded by it. There is a natural background of environmental radiation that we’re exposed to in the air, from common rocks and minerals around us, and from cosmic radiation from the Sun and stars. A small amount of radiation exposure is inevitable from our daily lives, and these small exposures pose miniscule risk in our lives. For perspective, a typical annual dose of naturally occurring radiation is about 0.3 rem, a very small amount! Figure 33.10 (source [PDF, 45 kB]) shows the annual average dose of different sources of radiation exposure (industrial and occupational doses are so small that they do not meaningfully show up on the graph). (Download this data [XLSX, 9 kB]) Radiation exposure from medical sources is only relevant for people undergoing these medical procedures. The dosage for a computed tomography (CT) scan, for example, is only received by the patient undergoing the scan.
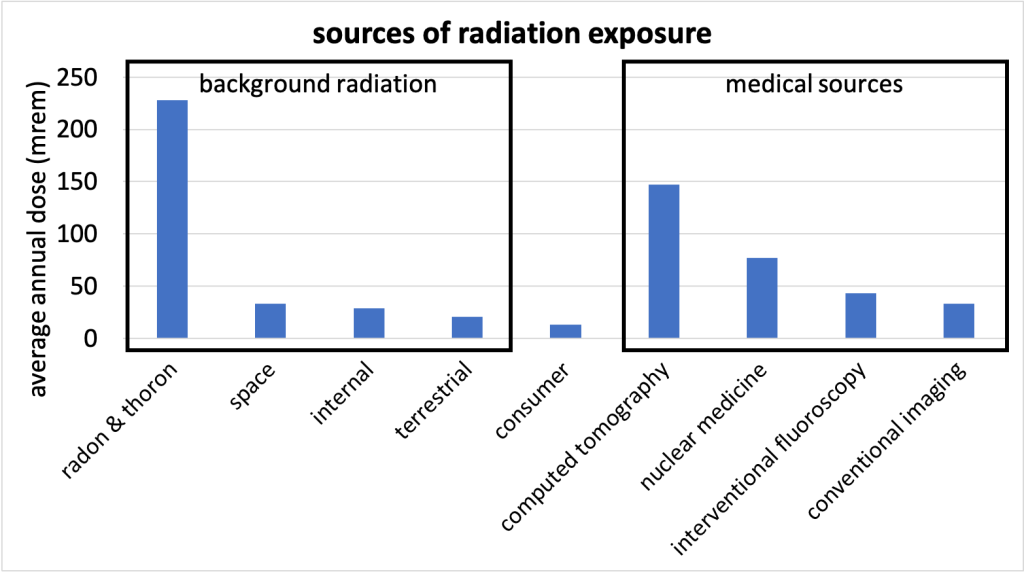
Medical diagnostics such as CT scans and nuclear medicine represent another source of possible radiation in our lives. The idea is that the benefit of the medical exposure to radiation outweighs the risk of the radiation exposure.
An isotope of radon, radon-222, is a heavy gas that may accumulate in basements that lack proper ventilation. High levels of radon-222 are dangerous, and radon detection and mitigation are both important to avoid unnecessary exposure to its alpha radiation.
There are other sources of environmental radiation. Historical atmospheric nuclear testing occurred between the 1940s and the 1960s. Thankfully, it was banned in 1963, so the dosage we receive from this historical time period is fortunately decreasing every day.
Occupational exposure is also possible. The National Council of Radiation Protection (NCRP) recommends that exposure not exceed 5 rem per year. Due to greater exposure to cosmic radiation at high altitudes, airline pilots and flight crews have limits on their annual flight hours. Other jobs that may encounter exposure to radiation will require employees to wear dosimeters to track their exposure. A collection of dosimeters hanging outside of the Advanced Photon Source at Argonne National Lab is shown in Figure 33.11.
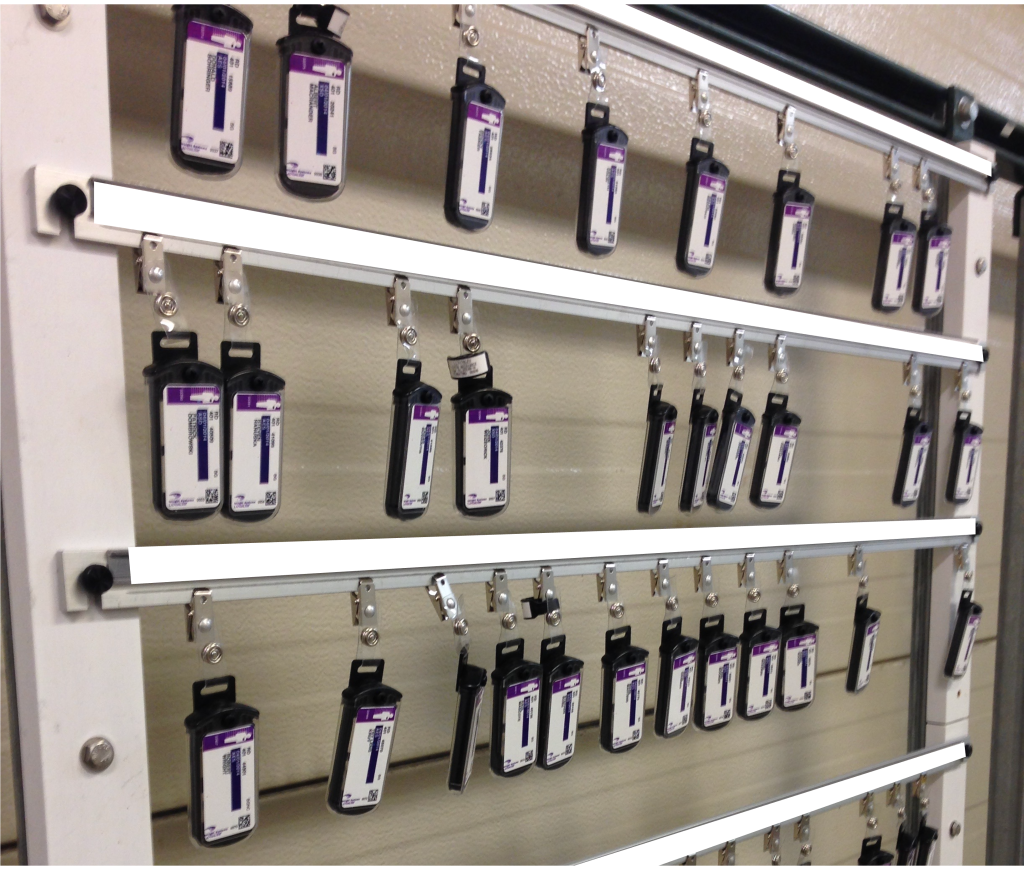
Burning coal, for example in power plants, leads to emission of radioactive thorium and uranium, and is a larger source of environmental exposure to radiation than nuclear power plants. Nuclear accidents (such as Three Mile Island, Chernobyl, and Fukushima) and nuclear waste are other sources of possible radiation exposure.
One of the best ways to reduce your exposure to radiation is to quit smoking if you’re a smoker. Cigarettes contain polonium-210, which undergoes alpha decay. When inhaled, it can represent a very large percentage of the annual radiation exposure of a smoker.
Artificial transmutation
The term transmutation refers to the change of one nuclide into a completely different nuclide. The processes of natural transmutation have been discussed: alpha and beta decay. (Recall that gamma decay does not change the isotope.) However, it is also possible to artificially transmute elements. Two particles can be smashed together to generate a new atomic nucleus.
For example, helium-4 and nitrogen-14 can be artificially transmuted to oxygen-17 and hydrogen-1 (a single proton):
Just as with natural transmutation, in the artificial transmutation process, atomic mass number and charge are conserved.
Long ago, alchemy was the study of transmuting elements such as lead into gold. It was hoped that a process could be found that would allow people to generate the precious metal.
Today, it is possible to use artificial transmutation to create gold. However, it is not remotely feasible economically. This is because the amount of energy that goes into the production of gold is enormous, and the gold that is created in the transmutation process would have to be the one isotope of gold that’s stable, otherwise it will naturally transmute into other elements.
Artificial transmutation has been used to generate atoms that don’t exist naturally. The so-called superheavy elements on the periodic table that have an atomic number greater than 103 have been created in laboratory settings. These isotopes have such short half-lives that if any natural sources existed (for example, as a result of a supernova), they have long since decayed. The creation and study of these elements leads to advances in physics and chemistry.
Artificial transmutation is also used in radiopharmacology to generate isotopes with short half-lives to use in medical treatment or diagnosis. Some elements such as technetium-99 need to be generated almost immediately before use, as the short half-life would otherwise cause them to decay almost entirely before their use.
Further reading
- Detection of xenon-124 decay – Researchers observed what they believe to be radioactive decay of xenon-124, which has a half-life of
years, one trillion times longer than the current age of the universe.
- What’s up with U? – This game from the National Museum of Nuclear Science and History explains the concept of half-life as well as the decay process of uranium-235.
- Radiation dose calculator – This online calculator from the American Nuclear Society estimates your average annual dose of radiation exposure.
- ORAU Museum of Radiation and Radioactivity – The website for the Oak Ridge Associated Universities Museum of Radiation and Radioactivity has a lot of information about radiation, radioactivity, and their history in the United States.
- Fiestaware – This webpage contains information about radioactive Fiesta dinnerware.
- Radioactivity in antiques – This website from the United States Environmental Protection Agency (EPA) describes different antique items that contain radioactive substances and emit alpha, beta, and/or gamma radiation.
Practice questions
Conceptual comprehension
- Americium-241, which is used in household smoke detectors, is produced from a plutonium isotope. What type of decay process must occur for plutonium to become americium?
- Consider two 100 g samples, one of nickel-63 (half-life: 100 years), and the other of nickel-56 (half-life: 6 days). Which sample will consist of more undecayed nickel isotopes after any given period of time (say, one week or one month)? Why is that?
Numerical analysis
- Determine the decay byproduct (the element, number of protons, and number of nucleons) of americium-241, which undergoes alpha decay.
- Determine the decay byproduct (the element, number of protons, and number of nucleons) of radium-223, which undergoes alpha decay.
- Determine the decay byproduct (the element, number of protons, and number of nucleons) of carbon-14, which undergoes beta-minus decay.
- What is the decay mechanism that occurs in each of the following decays:
- polonium-218 decays into astatine-218
- bismuth-210 decays into thallium-214
- aluminum-26 decays into magnesium-26
- nitrogen-11 decays into carbon-10
- Uranium-234 eventually decays into a stable isotope of lead. Which isotope of lead is the possible result: lead-206, lead-207, lead-208, or lead-209?
- In the previous question, uranium-234 decays into lead through a series of alpha and beta (minus) decays. How many alpha decays and how many beta-minus decays occur in this decay chain?
- Radium-223 has a half-life of 11.4 days. On average, how much radium-223 would be left in a 100 g sample after 22.8 days? 45.6 days?
- If a 100 g sample of an unknown radioactive element is left to decay for 8 days and, at that point, only contains 25% of the undecayed element, calculate the half-life of the element.
- If a flight crew receives 0.06 rem/hr on a long-haul polar route, calculate the maximum hours that the crew can fly annually in order to keep within the National Council of Radiation Protection recommendation of no more than 5 rem/yr of exposure.
Hands-on experiments
- You can simulate the radioactive decay process by shaking a box containing a large number of dice or pennies (that represent unstable nuclei) and removing those that come up a certain way (the decay products). For example, if you are shaking a box of pennies, you could remove all the pennies that come up tails after a shake and then continue with the remaining pennies until all of the pennies have “decayed.” Each shake represents a specific time interval.
- Start by placing a large number of pennies (or other coins) into a box. Securely fasten the lid. Shake the box vigorously for a couple of seconds. Open the box and remove all of the pennies that come up tails and set them aside. With the remaining coins left in the box, repeat the shaking process until you have no pennies left. How many time intervals does it take for the pennies to decay to half of their original value?
- Repeat the process using dice instead of pennies. Start by removing all of the dice that come up as 1. How many time intervals does it take for the dice to decay to half of their original value? How does this compare to the pennies?
- Repeat the process with dice, removing dice that come up as an odd number. How many time intervals does it take for the dice to decay to half of their original value? How does this compare to the pennies?
- Repeat the process with dice, removing dice that come up with a value between 1 and 5. How many time intervals does it take for the dice to decay to half of their original value? How does this compare to the pennies?
Electric charge is a fundamental property of matter that causes particles that carry a charge to experience a force when in the presence of an electric field.
Mass is a property of physical objects that relates to resistance to changes in motion: inertia. (symbol: m, unit: kg)
An electron is a fundamental building block of matter that has a negative charge and is found surrounding the nucleus of an atom.
The orbit of an electron describes the properties of the electron wave as it exists around the nucleus of an atom.
A proton is a subatomic particle that has a positive charge and resides in the nucleus of an atom.
A neutron is a subatomic particle that has no charge and resides in the nucleus of an atom.
A force is a push or a pull that causes an object to change its motion. More fundamentally, force is an interaction between two objects. (symbol: F, unit: N)
Electromagnetism describes the interactions that occur between charged objects.
Gravity is the attractive force experienced by objects of mass. It is one of the four fundamental forces.
An element is a substance that consists of atoms whose nuclei have the same atomic number. An element cannot be broken down further by chemical means.
The atomic number uniquely defines an element and is equal to the number of protons in atoms of that element. (symbol: Z)
Potential energy is energy that exists based on the arrangement or configuration of a substance.
A system is any collection of objects that we define. The concept of a system is used to determine what is internal or external when discussing concepts such as momentum conservation.
Elements that have the same number of protons but different numbers of neutrons are known as isotopes.
Energy is defined as the capability of an object (or collection of objects) to do useful work. (symbol: E, unit: J)
Radioactivity is the process by which an unstable nucleus releases energy and emits a particle.
Electron deexcitation is the process by which an electron loses energy and jumps down one or more energy levels. In the process, the electron either transfers energy to another particle, emits a photon, or generates heat.
Fission is the splitting of heavy atomic nuclei into lighter pieces.
An alpha particle is a helium nucleus (consisting of two protons and two neutrons).
Inertia is a property of matter whereby objects resist changes in their motion. Inertia is related to the mass of an object.
Path describes the total distance that an object travels as it moves from one point to another, measured along its trajectory. It is a scalar quantity. (symbols: d, x [for horizontal path], y [for vertical path], unit: m)
Centripetal force is a force, pointing toward the center of the rotational motion of an object, that causes an object to move in a circular path. (symbol: F_c, unit: N)
Atomic mass number is equal to the number of nucleons (protons and neutrons) in an atom. (symbol: A)
A nuclide is a nucleus of a particular isotope.
Condensation is the phase change from a gas to a liquid.
Energy levels describe the quantity of energy that each electron orbiting an atom can have. Each atom is uniquely defined by its discrete energy level values. The lowest energy level is known as the ground state.
Ionizing radiation refers to electromagnetic waves (UV, x-rays, and gamma rays) that contain sufficient energy to ionize atoms. These waves can cause damage to human DNA and other tissue.
An electromagnetic wave is a transverse wave that contains electric and magnetic fields oscillating at right angles to each other.
The electromagnetic spectrum describes the entire range of electromagnetic waves. Each subset of waves can be defined in different categories based on its frequency or wavelength.
A quark is a fundamental building block of matter, described by the standard model of physics. It is the building block of protons and neutrons.
Half-life is a description of the amount of time it takes, on average, for half of a particular unstable isotope to decay.
Gas is a state of matter where molecules are very free to move about and generally do not interact with each other except during collisions. This means that the shape and volume of a gas is free to change. Gas is one of the four most common phases of matter.
The term transmutation refers to the change of one nuclide into a completely different nuclide.
Physics is a branch of science that focuses on the fundamentals of the workings of our universe.