24. Magnetism
Summary
Magnetism
As we’ve learned, atoms contain a nucleus filled with protons and neutrons. Orbiting around the nucleus are electrons. The motion of these charged particles gives rise to magnetism. Most substances are not magnetic, which is because the magnetic effects of each atom cancel out, leading to an overall effect of no magnetism. When the magnetic effects of each atom don’t cancel out, the effect is a permanent magnet.
In some materials, the magnetic effects of individual atoms couple to those of neighboring atoms causing regions of atoms to align their internal magnets. Such regions are called magnetic domains. These microscopic regions may contain many billions of atoms. Each domain can then be thought of as a tiny permanent magnet. In an ordinary bar of iron, the individual domains are randomly oriented, so that ordinary bar of iron has no overall magnetic behavior. However, by placing the iron in a strong magnetic field or by stroking it in one direction with a magnet, the directions of each magnetic domain can be rotated into alignment. If the domains are somewhat aligned, the bar will become weakly magnetic. If all the domains are aligned in one direction, the iron bar will become strongly magnetic with opposite poles on each end. It is also possible to go the other way. If a magnet is heated, or damaged by getting repeatedly dropped (for example), it is possible for these domains to be knocked out of their alignment, and the magnet can become demagnetized.
For example, gadolinium is a metal that acts as a permanent magnet at temperatures less than about 20 oC. At room temperature, gadolinium will stick to magnetic surfaces, attract, and repel other magnets, as we would expect from a permanent magnet. This is demonstrated by Dr. Pasquale in the video below. At first, the piece of gadolinium is capable of attracting to another magnet. After heating the gadolinium with a heat gun, the magnetic domains become randomized, and the gadolinium is no longer magnetic. The gadolinium loses its magnetic attraction and gravity causes it to fall down. (The magnetic domains will become realigned after it cools back down again.)
Analogous to electrical interactions arising due to electric charges, magnetic interactions arise due to magnetic poles. The term poles was coined by Petrus Peregrinus after experimenting with magnets in the 13th century. In 1269, he carved a spherical magnet from an iron ore known as magnetite. Peregrinus noted that small pieces of iron placed on the sphere would align themselves along lines that pointed toward diametrically opposite sides of the sphere in much the same way that lines of longitude on the Earth meet at the geographic poles of the Earth. A magnetic pole is a region of a magnet where the forces involved in magnetic interactions are the strongest. Just as there are two types of charged particles (positive and negative), there are two types of magnetic poles: north and south. However, unlike electric charges, it is not possible to isolate an individual magnetic pole. Magnetic poles always come in opposite pairs. That is, every magnet contains both a north and a south pole.
Magnetic fields
The magnetic field describes the influence that a magnet has on other magnets and charged particles. We’ve described similar fields for gravity and electric charges. One of the interesting things about magnetic fields is that they can be easily visualized by using iron filings or a compass.
Iron filings, because they are magnetic, will align themselves along the magnetic field lines of a magnet. In Figure 24.1, iron filings are shown arranged around a bar magnet. (A bar magnet is a long rectangular type of magnet with opposite poles at each end; different types of magnets will be explained below.)
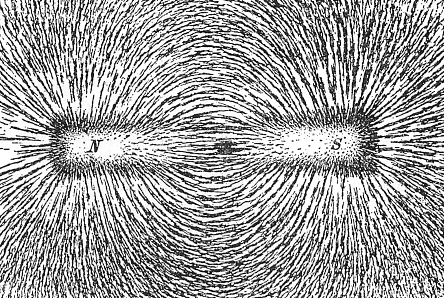
For a single bar magnet, the magnetic field loops around the magnet. The convention of a magnetic field is that, outside of the magnet, the field points from north to south pole. Inside the magnet, the field points from south to north.
A compass is a magnet balanced on its center of mass in a fluid or on a low friction pivot. That magnet is free to rotate, and will align itself with the strongest magnetic field in the vicinity. Compasses are used to navigate, as the Earth has a magnetic field (which will be discussed at the conclusion of this chapter). The painted end of a compass (which corresponds to the north pole of the compass) orients itself to point in a northerly direction on the surface of the Earth. This implies that the Earth itself behaves like a giant bar magnet.
In the video below, when a compass is held close to a magnet, the magnetic field of the magnet is strong enough to overcome the Earth’s magnetic field, and the painted end of the compass will point to the south pole of the magnet and away from the north pole of the magnet. We can see how the alignment of the compass changes as it is moved around a bar magnet.
Similarly to the attractive and repulsive forces involved with different types of charges (positive and negative), magnetic poles experience two types of interactions. Two like poles (north and north, or south and south) will repel. That is, if two like poles are held together, they will experience a force that causes them to move apart from each other. Two unlike poles (north and south) will attract. Therefore, if two unlike poles are held apart from each other, they will experience a force that causes them to come together. This is demonstrated in the video below, where Dr. Pasquale uses a low-friction turntable to allow magnets to freely rotate based on this force of magnetic attraction or repulsion. The north pole of a compass needle aligns itself in a northerly direction. If the north pole of the compass is attracted to the northern hemisphere of the Earth, then the pole in the northern hemisphere must actually be the south pole of Earth’s magnet.
Figure 24.2 depicts the magnetic field lines around attracting poles: a north and south pole. The magnetic field lines flow from one attracting pole to the next.
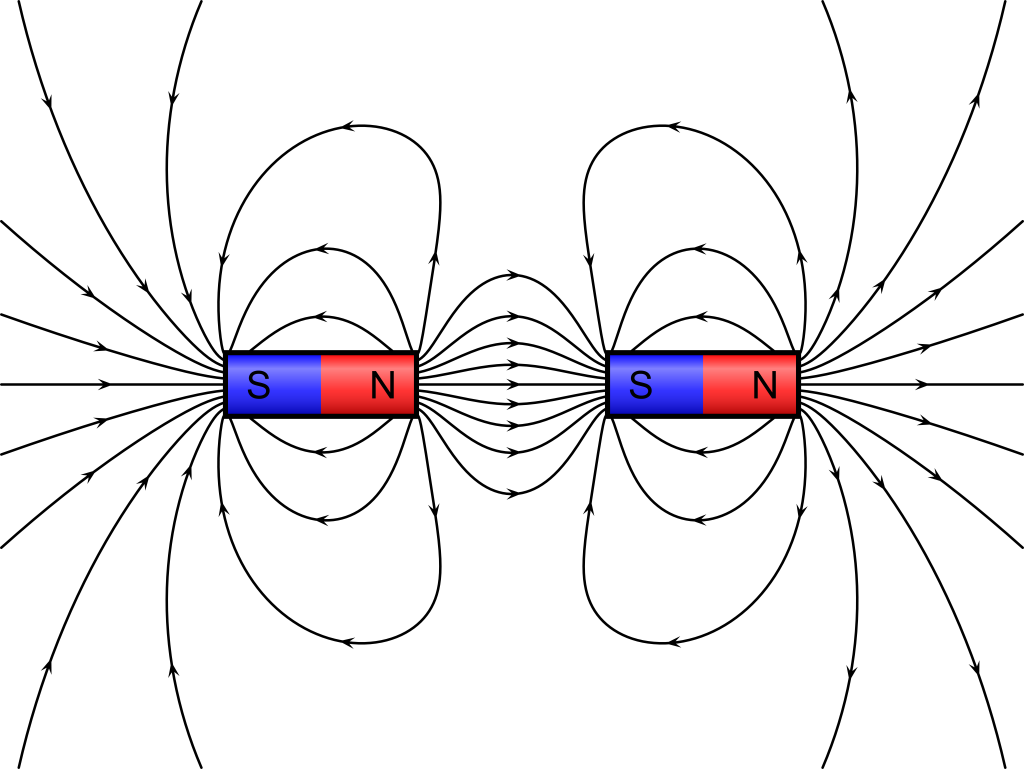
Figure 24.3 depicts the magnetic field lines around repelling poles: two north poles. The magnetic field lines flow away from the repelling pole.
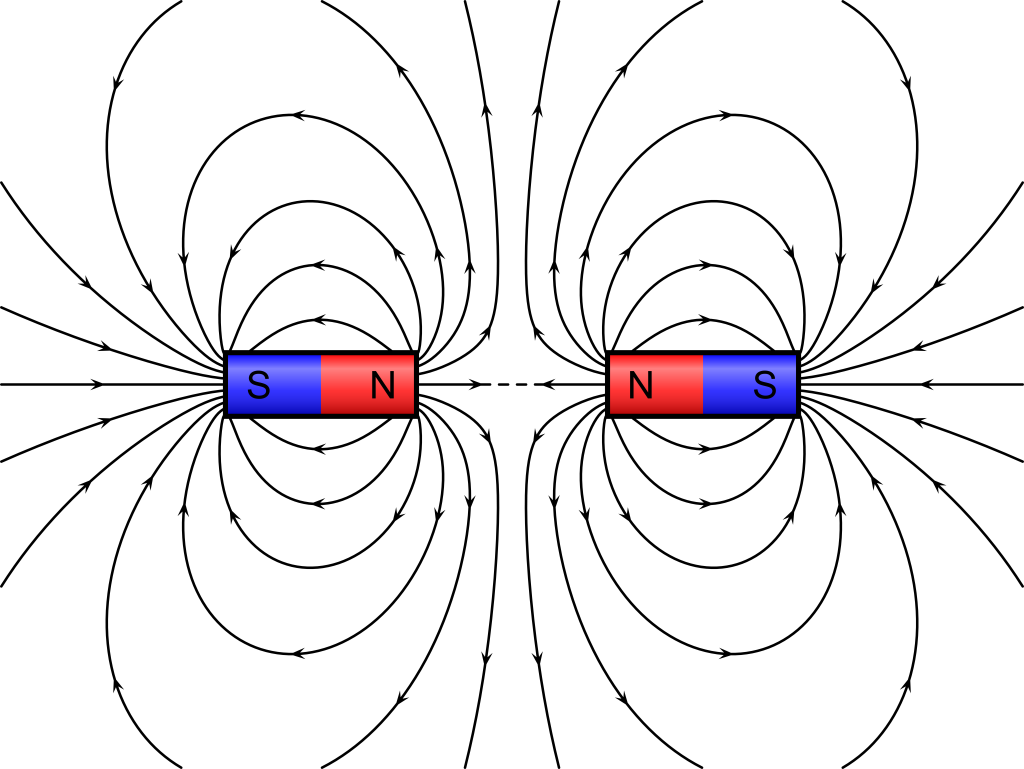
All magnets have both a north and a south pole. As far as physicists are currently aware, there is no way for a magnet to have only a north pole, or only a south pole. Magnetic poles always seem to exist in pairs. That is, the dipole (two equal and opposite poles) is the simplest magnetic structure. This leads to an interesting question: what happens if a magnet is broken in half?
In the video below, Dr. Pasquale has a bar magnet that contains a north and a south pole. When she splits it apart into two pieces, those two pieces each have their own north and south poles. Dr. Pasquale can prove that by using a compass to visualize the poles. This can additionally be demonstrated by showing the attraction and repulsion that exists between both magnet halves.
Types of magnets
Permanent magnets are made of different types of materials. Many low-cost magnets are made of iron oxide ceramics. Rare-earth metals are used to create very strong and small permanent magnets. Magnets also come in many different shapes and sizes. The videos used to demonstrate magnets in this chapter so far have used bar magnets. These are long rectangles with the north pole at one end, and the south pole at the other end.
A horseshoe magnet is essentially a bar magnet that has been bent into a U-shape. The north and south poles are still at opposite ends of the bar, but due to the bent shape, they are now located much closer together than they would be in a bar magnet. The resulting magnetic field is more concentrated in the region between the poles. The magnetic fields around a horseshoe magnet are depicted in Figure 24.4.
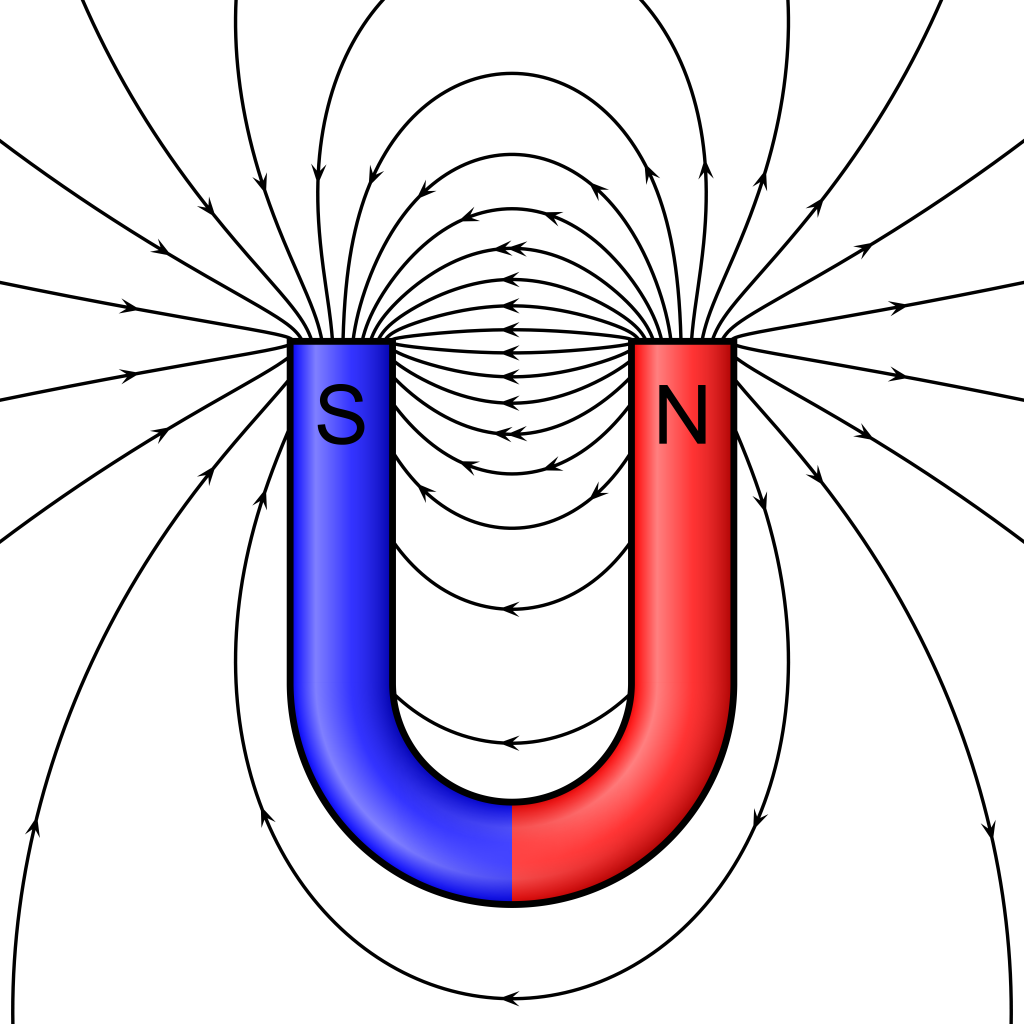
A disk magnet is a short cylindrical magnet. The poles of the disk magnet are located at the top and bottom surfaces of the disk. The magnetic fields around a disk magnet are depicted in Figure 24.5.
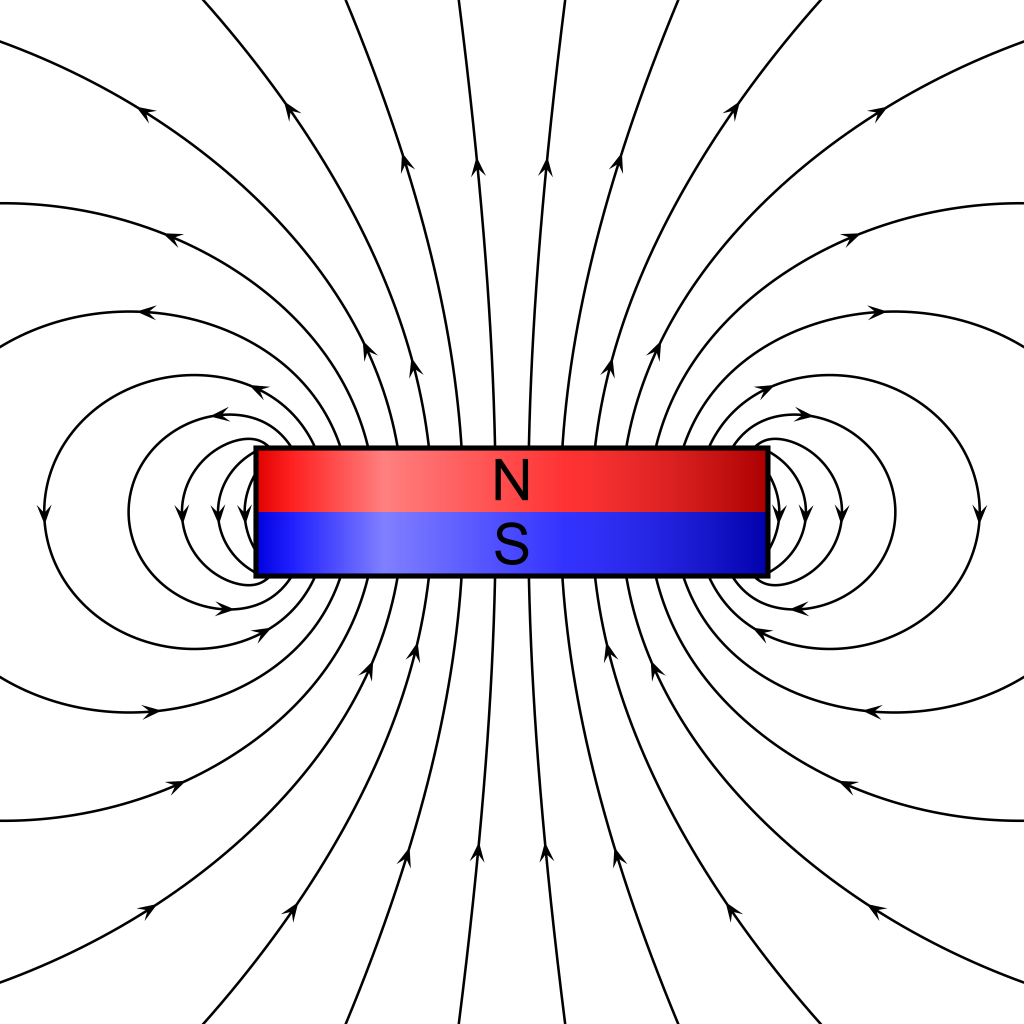
The poles of a disk magnet can be visualized by using a compass, shown in the video below.
Many rare-earth magnets, so named because they are made of rare-earth elements, are shaped into disk magnets. These rare-earth magnets are extremely strong, and care should be taken when handling them.
A ferrofluid is made up of liquid that can be attracted to a magnet. This is typically accomplished by suspending tiny coated magnetic particles in a liquid. Dr. Pasquale demonstrates the magnetic properties of a ferrofluid in the video below by holding a disk magnet near the ferrofluid.
Electromagnets
Permanent magnets have the advantage of being magnetic all the time, hence the name permanent magnet. However, sometimes there is a benefit in being able to control the magnetism of a material.
In 1820, the Danish physicist Hans Christian Oersted discovered (during a lecture demonstration, no less!) that current through a wire created a magnetic field. In the video below, Dr. Pasquale connected a wire to a battery through a switch. When the switch is open, no current flows through the wire. The compass points in its natural orientation. When Dr. Pasquale places the compass above the wire and closes the switch, the flow of current creates a magnetic field, causing the north pole of the compass to deflect. The direction of the north pole of the compass indicates the direction of that field. When she places the compass below the wire and closes the switch, we can see that the north pole of the compass points in the opposite direction, indicating that the magnetic field points in the opposite direction below the wire.
The magnetic field generated by a straight wire takes the shape of concentric circles around the wire. This means the field points in different directions above and below the wire. Figure 24.6 depicts this field for a wire viewed “head-on,” that is, with the wire depicted as perpendicular to the page (or screen, if you are reading this book on an electronic device). The current in this particular scenario is depicted as directed into the page. The direction of the magnetic field line curl can be determined by the “right-hand rule.” Using your right hand, point your thumb in the same direction as the current. Your fingers will then curl in the direction of the magnetic field around the wire. The direction of the magnetic field line curl will be in the opposite direction if the current flows out of the page.
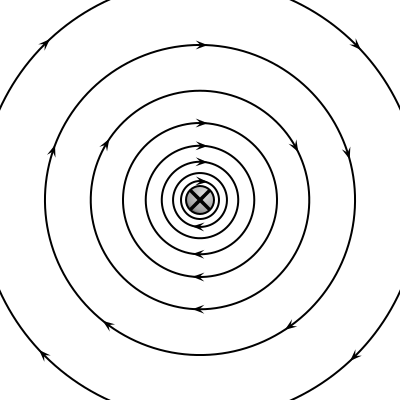
When the wire is coiled, it increases the strength of the magnetic field created by the current. This is because the coil consists of contributions of current from many hundreds of loops of wire. Dr. Pasquale demonstrates the magnetic field of a coil of wire in the video below. We can see how this field is stronger than the single straight wire by noticing how much more quickly the compass needle orients itself to the magnetic field for the coil as compared to the single wire.
To create a strong electromagnet, an iron core can be placed into the center of the coil of wire. The current creates a magnetic field that magnetizes the iron core. The magnetism of the iron core then further increases the magnetic field of the electromagnet. Dr. Pasquale demonstrates this magnetic field in the video below. The compass reacts to the magnetic field even faster after the iron core is placed into the center of the coiled wire.
An electromagnet can both attract and repel a bar magnet. Dr. Pasquale demonstrates this in the video below. This verifies the existence of both a north and a south pole in an electromagnet. We can determine which is which by seeing which end of the electromagnet attracts the north pole of a bar magnet, and which end of the electromagnet repels the north pole.
One of the major advantages of an electromagnet is that it can be turned on and off. Dr. Pasquale demonstrates this in the video below by using an electromagnet to pick up paper clips, move them, and then turn the electromagnet off by disconnecting the power source. Electromagnets built using this principle are used to lift and move around objects such as cars or scrap metal.
We saw in these video demonstrations that electricity can create magnetism. We’ll learn in the next chapter of this textbook that magnetism can create electricity!
The deflecting force
An electric charge moving in a magnetic field experiences a deflecting force. This interesting force is even more evidence linking electricity and magnetism.
The moving electric charge, which is simply an electric current, will experience a force whenever the current is not parallel to a magnetic field. This force will act perpendicularly to both the direction of current and the magnetic field. This is why it is called a deflecting force. This force is different from other forces we’ve learned about so far in this textbook, forces such as gravity and the Coulomb force that cause motion along the same direction as the field lines.
Because this is a perpendicular force, we can use the right-hand rule to determine the direction of magnetic force. First, use your index finger to point in the direction of the electric current. Next, use your middle finger to point in the direction of the magnetic field. (The magnetic field points from north to south outside of the magnet.) Point your thumb now perpendicular to both the direction of the current and the direction of the magnetic field. The direction that your thumb points indicates the direction of the deflecting force. This is shown in Figure 24.7. It should be emphasized that the magnetic field in this situation is from some external source. (It is not the magnetic field created by the current in the wire.)
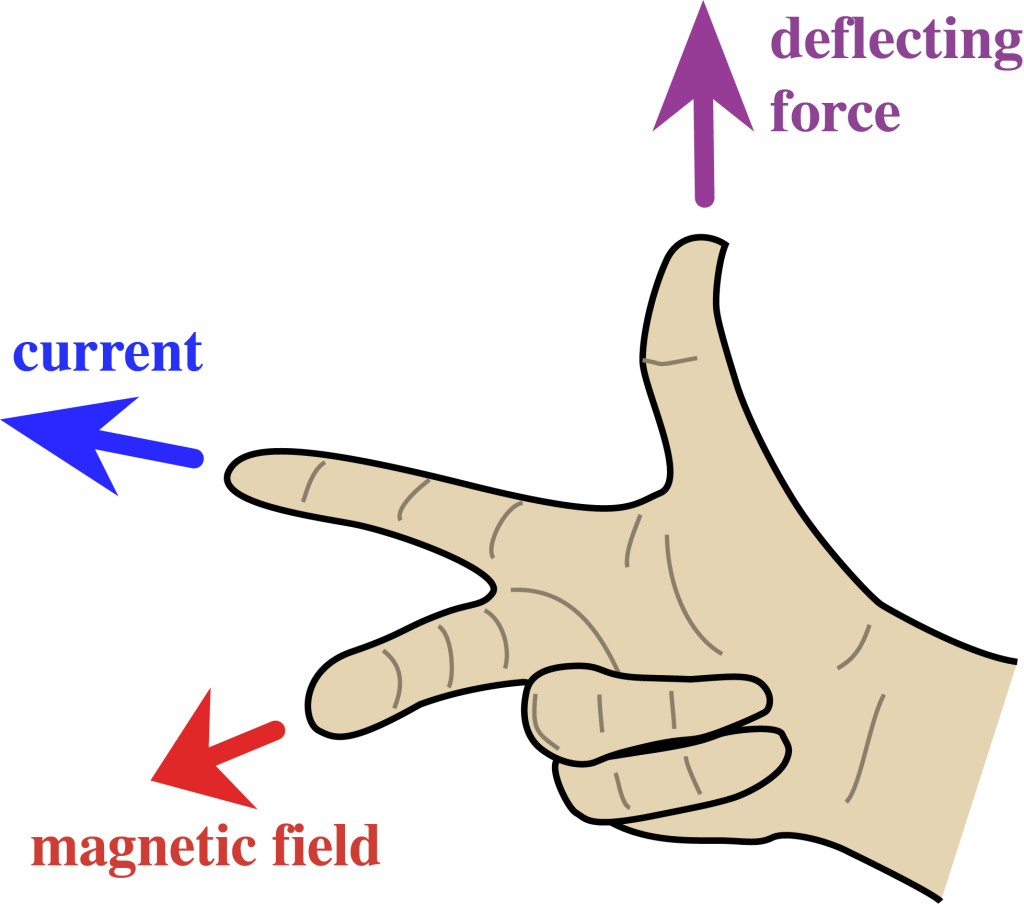
Let’s say the current points in the direction pointing out of the page and toward you. If the magnetic field points down, then the deflecting force will point to your right. If the current points out of the screen and toward you, and the magnetic field flips to point upward, then the deflecting force will point to your left. Four different scenarios of the magnetic deflection force are demonstrated in the video below.
The magnetic deflection force has many applications. A basic application is in motors. A direct current (DC) motor contains a battery, a coil of wire, and a magnet. The magnet creates a magnetic field, and the battery produces an electric current in the coil of wire. The deflecting forces produce a torque that causes the coil of wire to rotate, creating mechanical work. A DC motor is demonstrated in the video below.
The deflecting force is also used in circular particle accelerators. To cause any object to move in a circular path, there must be a centripetal force. That centripetal force can be caused by this magnetic deflecting force acting on charged particles. The Advanced Photon Source (seen in Figure 24.8) at Argonne National Lab in Lemont, Illinois, is a particle accelerator.
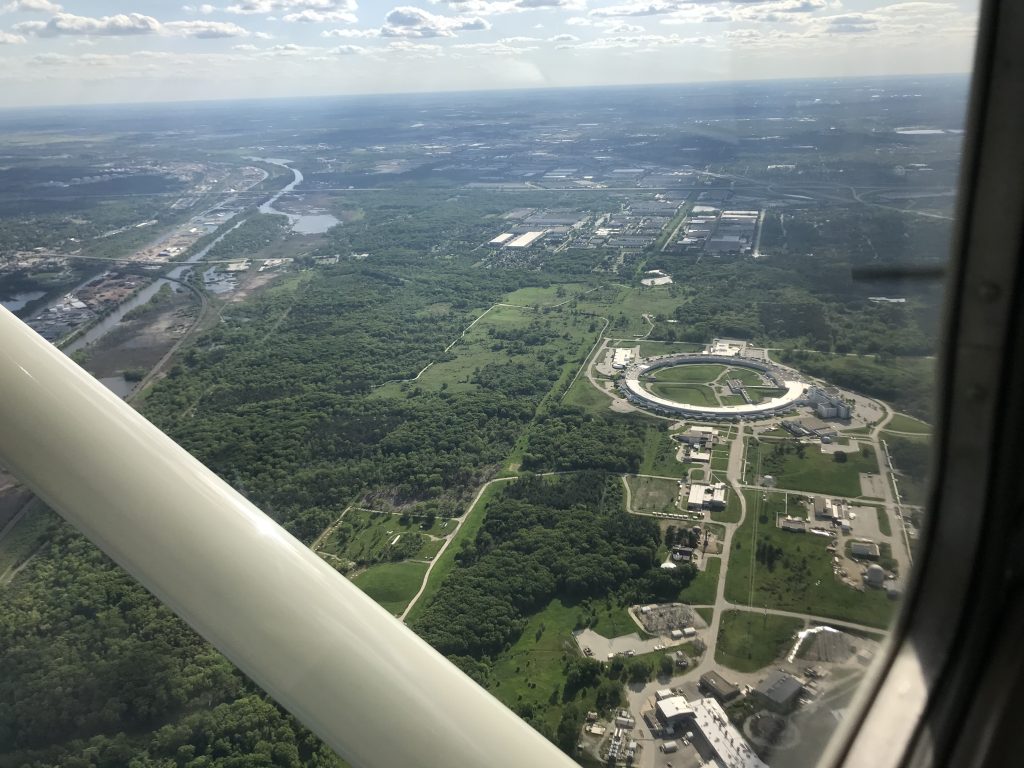
In France and Switzerland, the Large Hadron Collider is currently the world’s largest particle accelerator, at least at the time of this textbook writing. This particle accelerator is used to simulate conditions that existed just moments after the Big Bang and carry out advanced physics research to help scientists answer questions about how our universe works.
Earth’s magnetic field
As previously mentioned, if you wish to navigate without using GPS, a compass is a wonderful tool. The needle in a compass is a magnet, suspended in fluid, that will align itself to the strongest magnetic field in the vicinity. When outdoors, the north pole of a compass will align itself toward the north. Because opposite poles attract, the north pole of a compass attracting to the north indicates that the pole of the Earth in the northern hemisphere is actually the south pole of a magnet.
The Earth’s magnetic field is believed to be generated by the currents of molten iron and nickel in the outer core of the planet. Not only does this magnetic field allow us to navigate by use of compass, but it also protects the inhabitants of our planet from solar winds and space weather.
Solar wind consists of charged particles, emitted from the Sun, that would cause the Earth’s ozone layer to disintegrate. Without an ozone layer, we would not be protected from the Sun’s harmful UV rays, and would become more susceptible to skin cancer.
The Earth’s magnetic field deflects the solar wind, and while doing so, generates spectacular aurora (such as the aurora borealis, shown in Figure 24.9) in the process.
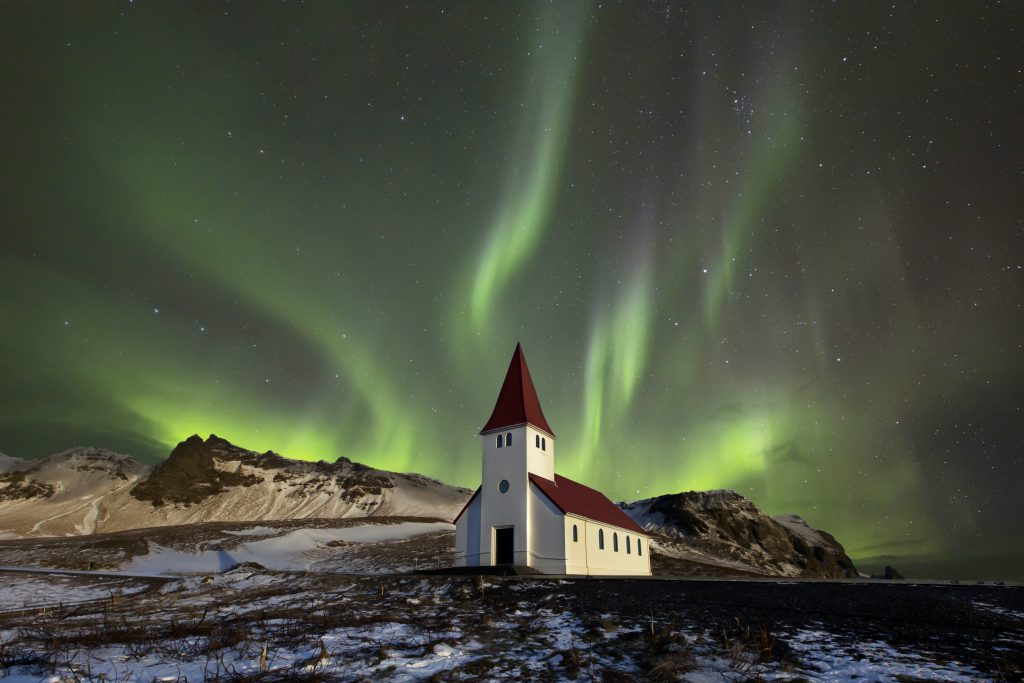
Further reading
- Magnetic Storm (Nova season 31, November 18, 2003) – This Nova episode gives a lot of information about the Earth’s magnetic field: why it’s important, and how it’s changed throughout the millennia.
- Ancient trees show when the Earth’s magnetic field last flipped out – This interesting news article from NPR gives some information about the last pole reversal, and how it was detected using trees.
- (Podcast) Magnets: the hidden objects powering your life – This 12-minute podcast gives a helpful introduction to magnets, magnetism, and why magnets are important.
Practice questions
Conceptual comprehension
- What are the benefits of permanent magnets over electromagnets?
- What are the benefits of electromagnets over permanent magnets?
- If the north pole of the magnet inside a compass points to the north pole of the Earth’s magnetic field, then what magnetic pole exists in the southern hemisphere of the Earth? Why?
Hands-on experiments
- Build your own compass. This document [PDF, 108 kB] contains the required materials and instructions.
- Build your own electromagnet. Using a nail, insulated copper wire, and a battery (such as a AA battery), wrap the copper wire around the nail and leave two loose ends. Connect the loose ends to the terminals of the battery. The nail becomes an electromagnet when the current flows through the wire. Test its magnetic strength by attracting small metal objects like paper clips. (Note: if you keep the wire connected directly to the battery for a long time, the current through the wire will eventually heat it up; to avoid this, disconnect the wire from the battery at intervals.)
A proton is a subatomic particle that has a positive charge and resides in the nucleus of an atom.
A neutron is a subatomic particle that has no charge and resides in the nucleus of an atom.
The orbit of an electron describes the properties of the electron wave as it exists around the nucleus of an atom.
An electron is a fundamental building block of matter that has a negative charge and is found surrounding the nucleus of an atom.
Electric charge is a fundamental property of matter that causes particles that carry a charge to experience a force when in the presence of an electric field.
Magnetism is a phenomenon that occurs when moving electric charges generate attractive and repulsive forces.
A magnetic domain is an area inside of a material where the magnetic effect of each individual atom couples to those of neighboring atoms, causing the internal magnets to be aligned.
Heat is energy that is transferred from one object to another in response to a difference in temperature. (symbol: Q, unit: cal)
Temperature defines the average kinetic energy of an object. It quantifies the “hotness” or “coldness” of something. (symbol: T, unit: °C or K)
Gravity is the attractive force experienced by objects of mass. It is one of the four fundamental forces.
A force is a push or a pull that causes an object to change its motion. More fundamentally, force is an interaction between two objects. (symbol: F, unit: N)
The center of mass is the point in an object (or system of objects) around which it rotates freely. It is considered to be the average position of all the mass in a system.
An element is a substance that consists of atoms whose nuclei have the same atomic number. An element cannot be broken down further by chemical means.
A liquid is a state of matter in which the constituent molecules will change their shape or arrangement but cannot be easily compressed to change their volume. Liquid is one of the four most common phases of matter.
Current describes the flow of electric charge through a circuit. (symbol: I, unit: A)
A motor converts electrical energy to mechanical work.
Direct current (DC) is a property of a voltage or current source in which the value of the voltage or current remains constant over time.
Torque is a twist or a turn that causes an object to change its rotational motion. Torque creates rotational acceleration and causes the rotation of an object to speed up, slow down, or change direction. (symbol: τ, unit: Nm)
Work is energy that is transferred to an object by exerting a force on that object over a distance. (symbol: W, unit: J)
Path describes the total distance that an object travels as it moves from one point to another, measured along its trajectory. It is a scalar quantity. (symbols: d, x [for horizontal path], y [for vertical path], unit: m)
Centripetal force is a force, pointing toward the center of the rotational motion of an object, that causes an object to move in a circular path. (symbol: F_c, unit: N)
Physics is a branch of science that focuses on the fundamentals of the workings of our universe.